D-Allulose 3-Epimerase of Bacillus Sp. Origin Manifests Profuse Heat
Total Page:16
File Type:pdf, Size:1020Kb
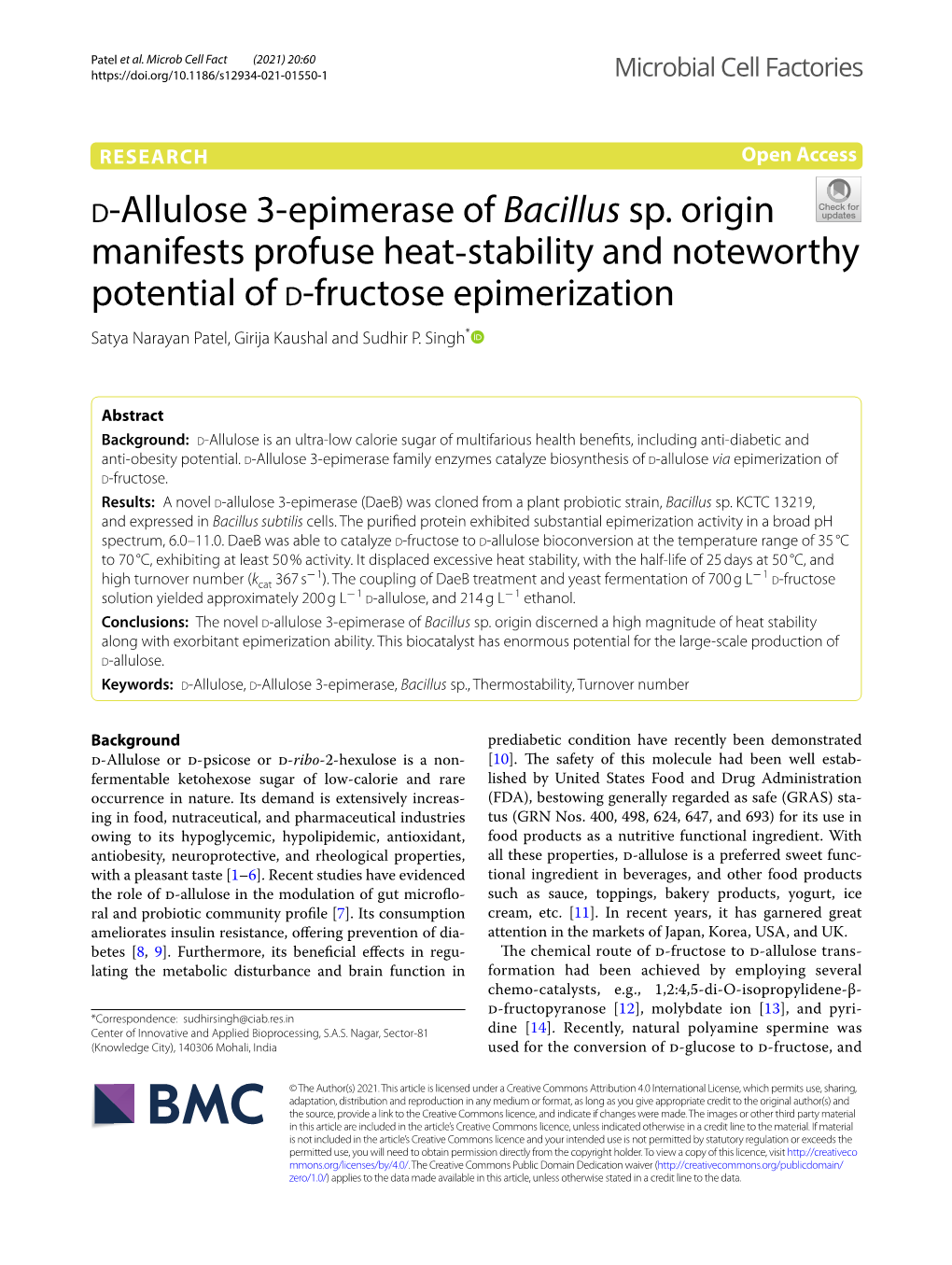
Load more
Recommended publications
-
Extremozymes of the Hot and Salty Halothermothrix Orenii
Extremozymes of the Hot and Salty Halothermothrix orenii Author Kori, Lokesh D Published 2012 Thesis Type Thesis (PhD Doctorate) School School of Biomolecular and Physical Sciences DOI https://doi.org/10.25904/1912/2191 Copyright Statement The author owns the copyright in this thesis, unless stated otherwise. Downloaded from http://hdl.handle.net/10072/366220 Griffith Research Online https://research-repository.griffith.edu.au Extremozymes of the hot and salty Halothermothrix orenii LOKESH D. KORI (M.Sc. Biotechnology) School of Biomolecular and Physical Sciences Science, Environment, Engineering and Technology Griffith University, Australia Submitted in fulfillment of the requirements of the degree of Doctor of Philosophy December 2011 STATEMENT OF ORIGINALITY STATEMENT OF ORIGINALITY This work has not previously been submitted for a degree or diploma in any university. To the best of my knowledge and belief, the thesis contains no material previously published or written by another person except where due reference is made in the thesis itself. LOKESH DULICHAND KORI II ACKNOWLEDGEMENTS ACKNOWLEDGEMENTS I owe my deepest gratitude to my supervisor Prof. Bharat Patel, for offering me an opportunity for being his postgraduate. His boundless knowledge motivates me for keep going and enjoy the essence of science. Without his guidance, great patience and advice, I could not finish my PhD program successfully. I take this opportunity to give my heartiest thanks to Assoc. Prof. Andreas Hofmann, (Structural Chemistry, Eskitis Institute for Cell & Molecular Therapies, Griffith University) for his support and encouragement for crystallographic work. I am grateful to him for teaching me about the protein structures, in silico analysis and their hidden chemistry. -
Developing a Genetic Manipulation System for the Antarctic Archaeon, Halorubrum Lacusprofundi: Investigating Acetamidase Gene Function
www.nature.com/scientificreports OPEN Developing a genetic manipulation system for the Antarctic archaeon, Halorubrum lacusprofundi: Received: 27 May 2016 Accepted: 16 September 2016 investigating acetamidase gene Published: 06 October 2016 function Y. Liao1, T. J. Williams1, J. C. Walsh2,3, M. Ji1, A. Poljak4, P. M. G. Curmi2, I. G. Duggin3 & R. Cavicchioli1 No systems have been reported for genetic manipulation of cold-adapted Archaea. Halorubrum lacusprofundi is an important member of Deep Lake, Antarctica (~10% of the population), and is amendable to laboratory cultivation. Here we report the development of a shuttle-vector and targeted gene-knockout system for this species. To investigate the function of acetamidase/formamidase genes, a class of genes not experimentally studied in Archaea, the acetamidase gene, amd3, was disrupted. The wild-type grew on acetamide as a sole source of carbon and nitrogen, but the mutant did not. Acetamidase/formamidase genes were found to form three distinct clades within a broad distribution of Archaea and Bacteria. Genes were present within lineages characterized by aerobic growth in low nutrient environments (e.g. haloarchaea, Starkeya) but absent from lineages containing anaerobes or facultative anaerobes (e.g. methanogens, Epsilonproteobacteria) or parasites of animals and plants (e.g. Chlamydiae). While acetamide is not a well characterized natural substrate, the build-up of plastic pollutants in the environment provides a potential source of introduced acetamide. In view of the extent and pattern of distribution of acetamidase/formamidase sequences within Archaea and Bacteria, we speculate that acetamide from plastics may promote the selection of amd/fmd genes in an increasing number of environmental microorganisms. -
Antonie Van Leeuwenhoek Journal of Microbiology
Antonie van Leeuwenhoek Journal of Microbiology Kroppenstedtia pulmonis sp. nov. and Kroppenstedtia sanguinis sp. nov., isolated from human patients --Manuscript Draft-- Manuscript Number: ANTO-D-15-00548R1 Full Title: Kroppenstedtia pulmonis sp. nov. and Kroppenstedtia sanguinis sp. nov., isolated from human patients Article Type: Original Article Keywords: Kroppenstedtia species, Kroppenstedtia pulmonis, Kroppenstedtia sanguinis, polyphasic taxonomy, 16S rRNA gene, thermoactinomycetes Corresponding Author: Melissa E Bell, MS Centers for Disease Control and Prevention Atlanta, Georgia UNITED STATES Corresponding Author Secondary Information: Corresponding Author's Institution: Centers for Disease Control and Prevention Corresponding Author's Secondary Institution: First Author: Melissa E Bell, MS First Author Secondary Information: Order of Authors: Melissa E Bell, MS Brent A. Lasker, PhD Hans-Peter Klenk, PhD Lesley Hoyles, PhD Catherine Spröer Peter Schumann June Brown Order of Authors Secondary Information: Funding Information: Abstract: Three human clinical strains (W9323T, X0209T and X0394) isolated from lung biopsy, blood and cerebral spinal fluid, respectively, were characterized using a polyphasic taxonomic approach. Comparative analysis of the 16S rRNA gene sequences showed the three strains belonged to two novel branches within the genus Kroppenstedtia: 16S rRNA gene sequence analysis of W9323T showed closest sequence similarity to Kroppenstedtia eburnea JFMB-ATE T (95.3 %), Kroppenstedtia guangzhouensis GD02T (94.7 %) and strain X0209T (94.6 %); sequence analysis of strain X0209T showed closest sequence similarity to K. eburnea JFMB-ATE T (96.4 %) and K. guangzhouensis GD02T (96.0 %). Strains X0209T and X0394 were 99.9 % similar to each other by 16S rRNA gene sequence analysis. The DNA-DNA relatedness was 94.6 %, confirming that X0209T and X0394 belong to the same species. -
Phylogenomic Analysis Supports the Ancestral Presence of LPS-Outer Membranes in the Firmicutes
Phylogenomic analysis supports the ancestral presence of LPS-outer membranes in the Firmicutes. Luisa Cs Antunes, Daniel Poppleton, Andreas Klingl, Alexis Criscuolo, Bruno Dupuy, Céline Brochier-Armanet, Christophe Beloin, Simonetta Gribaldo To cite this version: Luisa Cs Antunes, Daniel Poppleton, Andreas Klingl, Alexis Criscuolo, Bruno Dupuy, et al.. Phy- logenomic analysis supports the ancestral presence of LPS-outer membranes in the Firmicutes.. eLife, eLife Sciences Publication, 2016, 5, pp.e14589. 10.7554/eLife.14589.020. pasteur-01362343 HAL Id: pasteur-01362343 https://hal-pasteur.archives-ouvertes.fr/pasteur-01362343 Submitted on 8 Sep 2016 HAL is a multi-disciplinary open access L’archive ouverte pluridisciplinaire HAL, est archive for the deposit and dissemination of sci- destinée au dépôt et à la diffusion de documents entific research documents, whether they are pub- scientifiques de niveau recherche, publiés ou non, lished or not. The documents may come from émanant des établissements d’enseignement et de teaching and research institutions in France or recherche français ou étrangers, des laboratoires abroad, or from public or private research centers. publics ou privés. Distributed under a Creative Commons Attribution| 4.0 International License RESEARCH ARTICLE Phylogenomic analysis supports the ancestral presence of LPS-outer membranes in the Firmicutes Luisa CS Antunes1†, Daniel Poppleton1†, Andreas Klingl2, Alexis Criscuolo3, Bruno Dupuy4, Ce´ line Brochier-Armanet5, Christophe Beloin6, Simonetta Gribaldo1* 1Unite´ de -
Genome Diversity of Spore-Forming Firmicutes MICHAEL Y
Genome Diversity of Spore-Forming Firmicutes MICHAEL Y. GALPERIN National Center for Biotechnology Information, National Library of Medicine, National Institutes of Health, Bethesda, MD 20894 ABSTRACT Formation of heat-resistant endospores is a specific Vibrio subtilis (and also Vibrio bacillus), Ferdinand Cohn property of the members of the phylum Firmicutes (low-G+C assigned it to the genus Bacillus and family Bacillaceae, Gram-positive bacteria). It is found in representatives of four specifically noting the existence of heat-sensitive vegeta- different classes of Firmicutes, Bacilli, Clostridia, Erysipelotrichia, tive cells and heat-resistant endospores (see reference 1). and Negativicutes, which all encode similar sets of core sporulation fi proteins. Each of these classes also includes non-spore-forming Soon after that, Robert Koch identi ed Bacillus anthracis organisms that sometimes belong to the same genus or even as the causative agent of anthrax in cattle and the species as their spore-forming relatives. This chapter reviews the endospores as a means of the propagation of this orga- diversity of the members of phylum Firmicutes, its current taxon- nism among its hosts. In subsequent studies, the ability to omy, and the status of genome-sequencing projects for various form endospores, the specific purple staining by crystal subgroups within the phylum. It also discusses the evolution of the violet-iodine (Gram-positive staining, reflecting the pres- Firmicutes from their apparently spore-forming common ancestor ence of a thick peptidoglycan layer and the absence of and the independent loss of sporulation genes in several different lineages (staphylococci, streptococci, listeria, lactobacilli, an outer membrane), and the relatively low (typically ruminococci) in the course of their adaptation to the saprophytic less than 50%) molar fraction of guanine and cytosine lifestyle in a nutrient-rich environment. -
Complex Array of Endobionts in Petalomonas Sphagnophila, a Large Heterotrophic Euglenid Protist from Sphagnum-Dominated Peatlands
The ISME Journal (2010) 4, 1108–1120 & 2010 International Society for Microbial Ecology All rights reserved 1751-7362/10 www.nature.com/ismej ORIGINAL ARTICLE Complex array of endobionts in Petalomonas sphagnophila, a large heterotrophic euglenid protist from Sphagnum-dominated peatlands Eunsoo Kim1, Jong Soo Park2, Alastair GB Simpson2, Shigeru Matsunaga3, Masakatsu Watanabe3, Akio Murakami4, Katrin Sommerfeld1, Naoko T Onodera1 and John M Archibald1 1Department of Biochemistry and Molecular Biology, Canadian Institute for Advanced Research, Program in Integrated Microbial Biodiversity, Dalhousie University, Halifax, NS, Canada; 2Department of Biology, Canadian Institute for Advanced Research, Program in Integrated Microbial Biodiversity, Dalhousie University, Halifax, NS, Canada; 3Department of Photoscience, Graduate University for Advanced Sciences, Hayama, Japan and 4Kobe University Research Center for Inland Seas, Awaji, Japan Petalomonas sphagnophila is a poorly studied plastid-lacking euglenid flagellate living in Sphagnum-dominated peatlands. Here we present a broad-ranging microscopic, molecular and microspectrophotometric analysis of uncultured P. sphagnophila collected from four field locations in Nova Scotia, Canada. Consistent with its morphological characteristics, 18S ribosomal DNA (rDNA) phylogenies indicate that P. sphagnophila is specifically related to Petalomonas cantuscygni, the only other Petalomonas species sequenced to date. One of the peculiar characteristics of P. sphagnophila is the presence of several green-pigmented particles B5 lmin diameter in its cytoplasm, which a previously published study suggested to be cyanobacterial endosymbionts. New data presented here, however, suggest that the green intracellular body may not be a cyanobacterium but rather an uncharacterized prokaryote yet to be identified by molecular sequencing. 16S rDNA library sequencing and fluorescence in situ hybridizations show that P. -
Westminsterresearch Kroppenstedtia Pulmonis Sp. Nov. And
WestminsterResearch http://www.westminster.ac.uk/westminsterresearch Kroppenstedtia pulmonis sp. nov. and Kroppenstedtia sanguinis sp. nov., isolated from human patients Bell, M.E., Lasker, B.A., Klenk, H.-P., Hoyles, L., Spröer, C., Schumann, P. and Brown, J.M. This is an author's accepted manuscript of an article published in Antonie van Leeuwenhoek Journal of Microbiology, doi:10.1007/s10482-016-0663-z on 24/02/2016. The final publication is available at Springer via: https://dx.doi.org/10.1007/s10482-016-0663-z The WestminsterResearch online digital archive at the University of Westminster aims to make the research output of the University available to a wider audience. Copyright and Moral Rights remain with the authors and/or copyright owners. Whilst further distribution of specific materials from within this archive is forbidden, you may freely distribute the URL of WestminsterResearch: ((http://westminsterresearch.wmin.ac.uk/). In case of abuse or copyright appearing without permission e-mail [email protected] 1 Kroppenstedtia pulmonis sp. nov. and Kroppenstedtia sanguinis sp. nov., isolated from human patients Melissa E. Bell, Brent A. Lasker, Hans-Peter Klenk, Lesley Hoyles, Catherine Spröer, Peter Schumann, June M. Brown Keywords: Kroppenstedtia species, Kroppenstedtia pulmonis, Kroppenstedtia sanguinis, polyphasic taxonomy, 16S rRNA gene, thermoactinomycetes Corresponding Author M. E. Bell Centers for Disease Control and Prevention, Building 17, Room 2209, Mailstop D-11, 1600 Clifton Road, Atlanta, GA 30333, USA Phone: 404-639-1348 FAX: 404-639-3022 e-mail: [email protected] B. A. Lasker, J. M. Brown Bacterial Special Pathogens Branch, Division of High-Consequence Pathogens and Pathology, National Center for Emerging and Zoonotic Infectious Disease, Centers for Disease Control and Prevention, Atlanta, GA 30333, USA H.-P. -
Contents Topic 1. Introduction to Microbiology. the Subject and Tasks
Contents Topic 1. Introduction to microbiology. The subject and tasks of microbiology. A short historical essay………………………………………………………………5 Topic 2. Systematics and nomenclature of microorganisms……………………. 10 Topic 3. General characteristics of prokaryotic cells. Gram’s method ………...45 Topic 4. Principles of health protection and safety rules in the microbiological laboratory. Design, equipment, and working regimen of a microbiological laboratory………………………………………………………………………….162 Topic 5. Physiology of bacteria, fungi, viruses, mycoplasmas, rickettsia……...185 TOPIC 1. INTRODUCTION TO MICROBIOLOGY. THE SUBJECT AND TASKS OF MICROBIOLOGY. A SHORT HISTORICAL ESSAY. Contents 1. Subject, tasks and achievements of modern microbiology. 2. The role of microorganisms in human life. 3. Differentiation of microbiology in the industry. 4. Communication of microbiology with other sciences. 5. Periods in the development of microbiology. 6. The contribution of domestic scientists in the development of microbiology. 7. The value of microbiology in the system of training veterinarians. 8. Methods of studying microorganisms. Microbiology is a science, which study most shallow living creatures - microorganisms. Before inventing of microscope humanity was in dark about their existence. But during the centuries people could make use of processes vital activity of microbes for its needs. They could prepare a koumiss, alcohol, wine, vinegar, bread, and other products. During many centuries the nature of fermentations remained incomprehensible. Microbiology learns morphology, physiology, genetics and microorganisms systematization, their ecology and the other life forms. Specific Classes of Microorganisms Algae Protozoa Fungi (yeasts and molds) Bacteria Rickettsiae Viruses Prions The Microorganisms are extraordinarily widely spread in nature. They literally ubiquitous forward us from birth to our death. Daily, hourly we eat up thousands and thousands of microbes together with air, water, food. -
Identification of Bacteria Utilizing Biphenyl, Benzoate, and Naphthalene in Long-Term Contaminated Soil
Identification of Bacteria Utilizing Biphenyl, Benzoate, and Naphthalene in Long-Term Contaminated Soil Ondrej Uhlik1, Jiri Wald1, Michal Strejcek1, Lucie Musilova1, Jakub Ridl2, Miluse Hroudova2, Cestmir Vlcek2, Erick Cardenas3, Martina Mackova1, Tomas Macek1* 1 Department of Biochemistry and Microbiology, Faculty of Food and Biochemical Technology, Institute of Chemical Technology Prague, Prague, Czech Republic, 2 Department of Genomics and Bioinformatics, Institute of Molecular Genetics, Czech Academy of Sciences, Prague, Czech Republic, 3 Center for Microbial Ecology, Michigan State University, East Lansing, Michigan, United States of America Abstract Bacteria were identified associated with biodegradation of aromatic pollutants biphenyl, benzoate, and naphthalene in a long-term polychlorinated biphenyl- and polyaromatic hydrocarbon-contaminated soil. In order to avoid biases of culture- based approaches, stable isotope probing was applied in combination with sequence analysis of 16 S rRNA gene pyrotags amplified from 13C-enriched DNA fractions. Special attention was paid to pyrosequencing data analysis in order to eliminate the errors caused by either generation of amplicons (random errors caused by DNA polymerase, formation of chimeric sequences) or sequencing itself. Therefore, sample DNA was amplified, sequenced, and analyzed along with the DNA of a mock community constructed out of 8 bacterial strains. This warranted that appropriate tools and parameters were chosen for sequence data processing. 13C-labeled metagenomes isolated after the incubation of soil samples with all three studied aromatics were largely dominated by Proteobacteria, namely sequences clustering with the genera Rhodanobacter Burkholderia, Pandoraea, Dyella as well as some Rudaea- and Skermanella-related ones. Pseudomonads were mostly labeled by 13C from naphthalene and benzoate. The results of this study show that many biphenyl/benzoate-assimilating bacteria derive carbon also from naphthalene, pointing out broader biodegradation abilities of some soil microbiota. -
Reorganising the Order Bacillales Through Phylogenomics
Systematic and Applied Microbiology 42 (2019) 178–189 Contents lists available at ScienceDirect Systematic and Applied Microbiology jou rnal homepage: http://www.elsevier.com/locate/syapm Reorganising the order Bacillales through phylogenomics a,∗ b c Pieter De Maayer , Habibu Aliyu , Don A. Cowan a School of Molecular & Cell Biology, Faculty of Science, University of the Witwatersrand, South Africa b Technical Biology, Institute of Process Engineering in Life Sciences, Karlsruhe Institute of Technology, Germany c Centre for Microbial Ecology and Genomics, University of Pretoria, South Africa a r t i c l e i n f o a b s t r a c t Article history: Bacterial classification at higher taxonomic ranks such as the order and family levels is currently reliant Received 7 August 2018 on phylogenetic analysis of 16S rRNA and the presence of shared phenotypic characteristics. However, Received in revised form these may not be reflective of the true genotypic and phenotypic relationships of taxa. This is evident in 21 September 2018 the order Bacillales, members of which are defined as aerobic, spore-forming and rod-shaped bacteria. Accepted 18 October 2018 However, some taxa are anaerobic, asporogenic and coccoid. 16S rRNA gene phylogeny is also unable to elucidate the taxonomic positions of several families incertae sedis within this order. Whole genome- Keywords: based phylogenetic approaches may provide a more accurate means to resolve higher taxonomic levels. A Bacillales Lactobacillales suite of phylogenomic approaches were applied to re-evaluate the taxonomy of 80 representative taxa of Bacillaceae eight families (and six family incertae sedis taxa) within the order Bacillales. -
(ST). the Table
1 SUPPLEMENTAL MATERIALS Growth Media Modern Condition Seawater Freshwater Light T°C Atmosphere AMCONA medium BG11 medium – Synechococcus – – Synechocystis – [C] in PAL [C] in the [C] in the Gas Nutrients Modern Nutrients (ppm) Medium Medium Ocean NaNO3 CO2 ~407.8 Na2SO4 25.0mM 29mM Nitrogen 50 Standard (17.65mM) μmol (ST) NaNO NaNO 20°C O ~209’460 Nitrogen 3 3 MgSO 0.304mM photon 2 (549µM) (13.7µM) 4 /m2s FeCl3 6.56µM 2nM Ammonium 0.6g/L ZnSO 254nM 0.5nM ferric stock N ~780’790 4 2 citrate (10ml NaMoO 105nM 105nM 4 green stock/1L) 2 Table S 1 Description of the experimental condition defined as Standard Condition (ST). The table 3 shows the concentrations of fundamental elements, such as C, N, S, and Fe used for the AMCONA 4 seawater medium (Fanesi et al., 2014) and BG11 freshwater medium (Stanier et al., 1971) flushing air 5 with using air pump (KEDSUM-310 8W pump; Xiolan, China) Growth Media Possible Proterozoic T° Condition Modified Seawater Modified Freshwater Light Atmosphere AMCONA medium BG11 medium C – Synechococcus – – Synechocystis – [C] in [C] in PPr Nutrients PPr Nutrients Medium Gas ppm Medium NH Cl Na SO 3mM Nitrogen 4 3 2 4 (0.0035mM) CO 2 10’000ppm (20%) NH Cl Possible (~ 2’450% Nitrogen 4 3 MgSO 0.035mM 50 with 20ml/ (100µM) 4 Proterozoic PAL) μmol 20° min (PPr) photon C O2 20’000ppm /m2s (in Air) (~ 10% FeCl 200nM with 5ml/ 3 PAL) Ammonium 0.6g/L stock min ferric 10ml N ZnSO 0.0nM 2 4 citrate green stock/1L (100%) Base gas with NaMoO4 10.5nM 200ml/min 6 Table S 2 Description of the experimental condition defined as Possible Proterozoic Condition (PPr). -
Kroppenstedtia Eburnea Gen. Nov., Sp. Nov., A
International Journal of Systematic and Evolutionary Microbiology (2011), 61, 2304–2310 DOI 10.1099/ijs.0.026179-0 Kroppenstedtia eburnea gen. nov., sp. nov., a thermoactinomycete isolated by environmental screening, and emended description of the family Thermoactinomycetaceae Matsuo et al. 2006 emend. Yassin et al. 2009 Mathias von Jan,1 Nicole Riegger,2 Gabriele Po¨tter,1 Peter Schumann,1 Susanne Verbarg,1 Cathrin Spro¨er,1 Manfred Rohde,3 Bettina Lauer,2 David P. Labeda4 and Hans-Peter Klenk1 Correspondence 1DSMZ – German Collection of Microorganisms and Cell Cultures, 38124 Braunschweig, Germany Hans-Peter Klenk 2Microbiology, Vetter Pharma-Fertigung GmbH & Co. KG, 88212 Ravensburg, Germany [email protected] 3HZI – Helmholtz Centre for Infection Research, 38124 Braunschweig, Germany 4National Center for Agricultural Utilization Research, USDA-ARS, Peoria, IL 61604, USA A Gram-positive, spore-forming, aerobic, filamentous bacterium, strain JFMB-ATET, was isolated in 2008 during environmental screening of a plastic surface in grade C in a contract manufacturing organization in southern Germany. The isolate grew at temperatures of 25–50 6C and at pH 5.0–8.5, forming ivory-coloured colonies with sparse white aerial mycelia. Chemotaxonomic and molecular characteristics of the isolate matched those described for members of the family Thermoactinomycetaceae, except that the cell-wall peptidoglycan contained LL-diaminopimelic acid, while all previously described members of this family display this diagnostic diamino acid in meso-conformation. The DNA G+C content of the novel strain was 54.6 mol%, the main polar lipids were diphosphatidylglycerol, phosphatidylethanolamine and phosphatidylglycerol, and the major menaquinone was MK-7. The major fatty acids had saturated C14–C16 branched chains.