Neural Mechanisms Involved in Enterotoxin- Induced Intestinal Hypersecretion
Total Page:16
File Type:pdf, Size:1020Kb
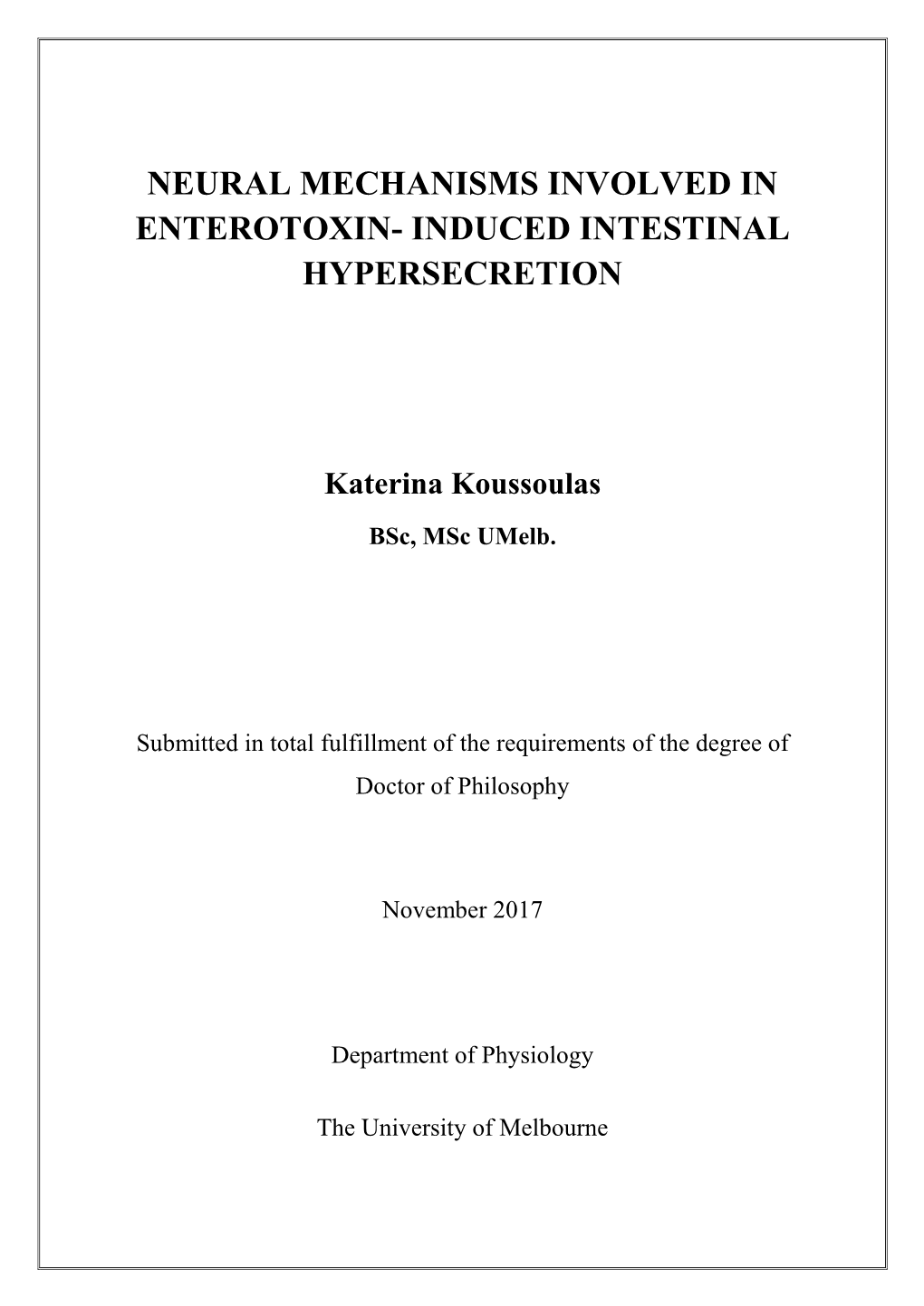
Load more
Recommended publications
-
Gastrointestinal Motility
Gastrointestinal Motility H. J. Ehrlein and M.Schemann 1. Motility of the stomach Anatomic regions of the stomach are the fundus, corpus (body), antrum and pylorus. The functional regions of the stomach do not correspond to the anatomic regions. Functionally, the stomach can be divided into the gastric reservoir and the gastric pump (Fig. 1). The gastric reservoir consists of the fundus and corpus. The gastric pump is represented by the area at which peristaltic waves occur: it includes the distal part of the corpus and the antrum. Due to different properties of the smooth muscle cells the gastric reservoir is characterised by tonic activity and the gastric pump by phasic activity. AB Gastric reservoir Fundus tonic contractions Pylorus Corpus Antrum Gastric pump phasic contractions Figure 1 . The stomach can be divided into three anatomic (A) and two functional regions (B) 1.1 Function of the gastric reservoir At the beginning of the 20 th century it was already observed that with increasing volume of the stomach the internal pressure of the stomach increases only slightly. In dogs, for instance, the increase in pressure is only 1.2 cm of water/100 ml volume. The small increase in gastric pressure indicates that the stomach does not behave like an elastic balloon but that it relaxes as it fills. Three kinds of gastric relaxation can be differentiated: a receptive, an adaptive and a feedback-relaxation of the gastric reservoir. The receptive relaxation consists of a brief relaxation during chewing and swallowing. The stimulation of mechano-receptors in the mouth and pharynx induces vago-vagal reflexes which cause a relaxation of the gastric reservoir (Fig. -
Octreotide in Gastrointestinal Motility Disorders Gut: First Published As 10.1136/Gut.35.3 Suppl.S11 on 1 January 1994
Gut 1994; supplement 3: S11 -S 14 Sll Octreotide in gastrointestinal motility disorders Gut: first published as 10.1136/gut.35.3_Suppl.S11 on 1 January 1994. Downloaded from C Owyang Abstract Intestinal effects of octreotide in The effects of octreotide on six normal scleroderma subjects and five patients with sclero- About 50% of patients with scleroderma have derma were investigated. Changes in small bowel dysfunction.5 In such patients, intestinal motility and in plasma motilin manometry shows patterns (known as the were examined after a single injection of migrating motor complex) in the small bowel octreotide. Octreotide stimulated intense during fasting6 and this may be clinically intestinal motor activity in normal sub- manifested as intestinal pseudo-obstruction jects. Motility patterns in the scleroderma and bacterial overgrowth. These problems are patients were chaotic and non-pro- difficult to treat because standard stimulatory pagative, but, after octreotide was given, prokinetic agents are not effective in sclero- became well coordinated, aborally derma.6 We therefore recently undertook a directed, and nearly as intense as in study to determine the effects of octreotide in normal volunteers. Clinical responses and six normal subjects and in five patients with changes in breath hydrogen were also scleroderma who had abdominal pain, nausea, evaluated in the five scleroderma patients bloating, and a change in intestinal contrac- who had further treatment with octreotide tility.7 We examined the changes in intestinal at a dose of 50 pugtday subcutaneously for motility and in plasma motilin, a gastrointesti- three weeks. A reduction in symptoms of nal hormone that stimulates intestinal motor abdominal pain, nausea, vomiting, and activity, after single injections of octreotide bloating was seen. -
5-Hydroxytryptamine Andhuman Small Intestinal Motility
496 Gut 1994; 35:496-500 5-Hydroxytryptamine and human small intestinal motility: effect ofinhibiting 5-hydroxytryptamine reuptake D A Gorard, G W Libby, M J G Farthing Abstract nature is poorly understood.'0 In animals, Parenteral 5-hydroxytryptamine stimulates migrating motor complex cycling can be small intestinal motility, but the effect of con- modified by administration of 5-HT, its pre- tinuous stimulation with 5-hydroxytryptamine cursor 5-hydroxytryptophan, and its antago- on the human migrating motor complex is nists." '4 The effect of 5-HT on the migrating unknown. Using a selective 5-hydroxytrypta- motor complex in humans has not been studied. mine reuptake inhibitor, paroxetine, this study Tachyphylaxis to 5-HT given intravenously,45 investigated the effect of indirect 5-hydroxy- and associated cardiovascular and pulmonary tryptamine agonism on fasting small responses limit prolonged infusion of 5-HT in intestinal motility and transit. Eight healthy humans. The effect, however, of 5-HT agonism subjects were studied while receiving paroxe- on human small intestinal motor function might tine 30 mg daily for five days and while receiv- be alternatively investigated using a selective ing no treatment, in random order. Ambulant 5-HT reuptake inhibitor. Paroxetine selectively small intestinal motility was recorded from five inhibits the neuronal reuptake of 5-HT, increas- sensors positioned from the duodenojejunal ing the availability of synaptic 5-HT. Its ability flexure to the ileum for 16-18 hours. Paroxe- to inhibit 5-HT reuptake exceeds its ability to tine reduced the migrating motor complex inhibit noradrenaline reuptake by a factor of periodicity mean (SEM) from 81 (6) min to 67 320," making it four to five hundred times (4) min (p<005), and increased the propaga- as selective as the standard tricycic anti- tion velocity of phase III from 3-1 to 4-7 cm/ depressants imipramine and amitriptyline. -
Aandp2ch25lecture.Pdf
Chapter 25 Lecture Outline See separate PowerPoint slides for all figures and tables pre- inserted into PowerPoint without notes. Copyright © McGraw-Hill Education. Permission required for reproduction or display. 1 Introduction • Most nutrients we eat cannot be used in existing form – Must be broken down into smaller components before body can make use of them • Digestive system—acts as a disassembly line – To break down nutrients into forms that can be used by the body – To absorb them so they can be distributed to the tissues • Gastroenterology—the study of the digestive tract and the diagnosis and treatment of its disorders 25-2 General Anatomy and Digestive Processes • Expected Learning Outcomes – List the functions and major physiological processes of the digestive system. – Distinguish between mechanical and chemical digestion. – Describe the basic chemical process underlying all chemical digestion, and name the major substrates and products of this process. 25-3 General Anatomy and Digestive Processes (Continued) – List the regions of the digestive tract and the accessory organs of the digestive system. – Identify the layers of the digestive tract and describe its relationship to the peritoneum. – Describe the general neural and chemical controls over digestive function. 25-4 Digestive Function • Digestive system—organ system that processes food, extracts nutrients, and eliminates residue • Five stages of digestion – Ingestion: selective intake of food – Digestion: mechanical and chemical breakdown of food into a form usable by -
Microscale Bioreactors for in Situ Characterization of GI Epithelial Cell Physiology Received: 7 February 2017 Cait M
www.nature.com/scientificreports OPEN Microscale Bioreactors for in situ characterization of GI epithelial cell physiology Received: 7 February 2017 Cait M. Costello1, Mikkel B. Phillipsen1, Leonard M. Hartmanis1, Marek A. Kwasnica1, Victor Accepted: 14 September 2017 Chen1, David Hackam2, Matthew W. Chang3, William E. Bentley4 & John C. March1 Published: xx xx xxxx The development of in vitro artifcial small intestines that realistically mimic in vivo systems will enable vast improvement of our understanding of the human gut and its impact on human health. Synthetic in vitro models can control specifc parameters, including (but not limited to) cell types, fuid fow, nutrient profles and gaseous exchange. They are also “open” systems, enabling access to chemical and physiological information. In this work, we demonstrate the importance of gut surface topography and fuid fow dynamics which are shown to impact epithelial cell growth, proliferation and intestinal cell function. We have constructed a small intestinal bioreactor using 3-D printing and polymeric scafolds that mimic the 3-D topography of the intestine and its fuid fow. Our results indicate that TEER measurements, which are typically high in static 2-D Transwell apparatuses, is lower in the presence of liquid sheer and 3-D topography compared to a fat scafold and static conditions. There was also increased cell proliferation and discovered localized regions of elevated apoptosis, specifcally at the tips of the villi, where there is highest sheer. Similarly, glucose was actively transported (as opposed to passive) and at higher rates under fow. Immortal intestinal epithelial cell lines such as Caco-2 are useful for in vitro studies of intestinal function, as they have ability to form polarized monolayers on membranes that separate the apical and basolateral space. -
Mechanism of Interdigestive Migrating Motor Complex
J Neurogastroenterol Motil, Vol. 18 No. 3 July, 2012 pISSN: 2093-0879 eISSN: 2093-0887 http://dx.doi.org/10.5056/jnm.2012.18.3.246 Review JNM Journal of Neurogastroenterology and Motility Mechanism of Interdigestive Migrating Motor Complex Toku Takahashi Department of Surgery, Medical College of Wisconsin and Zablocki VA Medical Center, Milwaukee, Wisconsin, USA Migrating motor complex (MMC) is well characterized by the appearance of gastrointestinal contractions in the interdigestive state. This review article discussed the mechanism of gastrointestinal MMC. Luminal administration of 5-hydroxytryptamine (5-HT) initiates duodenal phase II followed by gastrointestinal phase III with a concomitant increase of plasma motilin release in conscious dogs. Duodenal 5-HT concentration is increased during gastric phase II and phase III. Intravenous infusion of mo- tilin increases luminal 5-HT content and induces gastrointestinal phase III. 5-HT4 antagonists significantly inhibits both of gas- tric and intestinal phase III, while 5-HT3 antagonists inhibited only gastric phase III. These suggest that gastrointestinal MMC cycle is mediated via the interaction between motilin and 5-HT by the positive feedback mechanism. Gastric MMC is regulated via vagus, 5-HT3/4 receptors and motilin, while intestinal MMC is regulated via intrinsic primary afferent neurons and 5-HT4 receptors. Stress is highly associated with the pathogenesis of functional dyspepsia. Acoustic stress attenuates gastric phase III without affecting intestinal phase III in conscious dogs, via reduced vagal activity and increased sympathetic activity. It has been shown that subset of functional dyspepsia patients show reduced vagal activity and impaired gastric phase III. The phys- iological importance of gastric MMC is a mechanical and chemical cleansing of the empty stomach in preparation for the next meal. -
Signaling in Enteric Glia Drives Neural Programs That Regulate Intestinal Motility in Mice Jonathon L
ORIGINAL RESEARCH Agonist-Evoked Ca2D Signaling in Enteric Glia Drives Neural Programs That Regulate Intestinal Motility in Mice Jonathon L. McClain,1,* David E. Fried,1,* and Brian D. Gulbransen1,2 1Department of Physiology and 2Neuroscience Program, Michigan State University, East Lansing, Michigan SUMMARY ntestinal functions such as peristalsis require the coor- I dinated activity of multiple cell types throughout the gut Enteric glia monitor neurotransmission in the gut but the wall, including enteroendocrine cells, nerves, interstitial cells, significance of glial activity has remained unclear. We found and smooth muscle.1 Yet deciphering the exact contribution that selectively triggering glial activity has the potential to of individual cell types is extremely challenging because of drive excitatory neural programs that control gut motility. the complex nature of tissue and the overlapping expression of many signaling molecules. Each cell type in the chain of command from mucosa to smooth muscle is clearly BACKGROUND & AIMS: Gastrointestinal motility is regulated essential for the transduction of luminal cues into motor by enteric neural circuitry that includes enteric neurons and responses, and the ultimate integration and execution of mo- glia. Enteric glia monitor synaptic activity and exhibit responses tor patterns depends on the neural circuitry within the enteric to neurotransmitters that are encoded by intracellular calcium nervous system (ENS).2 However, the exact roles of many þ (Ca2 ) signaling. What role evoked glial responses play in the classes of cells in the gut wall is still highly debated.3–5 neural regulation of gut motility is unknown. We tested how One class of cells that have gained particular interest 2þ evoking Ca signaling in enteric glia affects the neural control recently are the enteric glia.5 These astrocyte-like periph- of intestinal motility. -
Pentagastrin Induced Motility Pattern in the Human Upper Gastrointestinal Tract Is Reversed by Proglumide
Gut: first published as 10.1136/gut.25.9.953 on 1 September 1984. Downloaded from Gut, 1984, 25, 953-956 Pentagastrin induced motility pattern in the human upper gastrointestinal tract is reversed by proglumide J F ERCKENBRECHT, J CASPARI, AND M WIENBECK From the Department of Internal Medicine D, University of Dusseldorf, Dusseldorf, FR Germany SUMMARY The effects of pentagastrin and the putative gastrin antagonist proglumide on interdigestive motility of the upper small bowel were studied in a randomised double blind study in 10 healthy human volunteers. Intraluminal pressures were recorded manometrically in the duodenum and jejunum for five hours. Sixty minutes after starting a pentagastrin infusion (0.15 ,ug/kg/h) either placebo or proglumide was infused intravenously. Pentagastrin converted the normal interdigestive motility to irregular motor activity, while proglumide restored the periodic fasted pattern. We conclude that gastrin is a likely candidate involved in the conversion of the fasted to the fed motility pattern in the human upper gut. In the fasted state, motility of the stomach and small Methods intestine of man is characterised by periodic activity of the interdigestive migrating motor complex SUBJECTS (MMC). 1-4 Immediately after feeding, gastro- Ten healthy male volunteers (mean age 25±2 intestinal motility becomes irregular.4 In the con- (x ± SD) years) were examined in a double blind, version of the interdigestive to the digestive motility randomised, crossover study after giving informed pattern not only neural mechanisms,5 but also consent. http://gut.bmj.com/ gastrointestinal hormones, particularly chole- Intraluminal pressures were measured mano- cystokinin (CCK) and gastrin, appear to be metrically by a triple lumen polyvinyl tube. -
Fundamentals of the Gut for Capsule Engineers
This is a repository copy of Fundamentals of the gut for capsule engineers. White Rose Research Online URL for this paper: https://eprints.whiterose.ac.uk/164293/ Version: Accepted Version Article: Barducci, L, Norton, J, Sarker, S et al. (4 more authors) (2020) Fundamentals of the gut for capsule engineers. Progress in Biomedical Engineering. ISSN 2516-1091 https://doi.org/10.1088/2516-1091/abab4c © 2020 IOP Publishing Ltd. This is an author accepted version of an article published in Progress in Biomedical Engineering. Uploaded in accordance with the publisher's self- archiving policy. Reuse This article is distributed under the terms of the Creative Commons Attribution-NonCommercial-NoDerivs (CC BY-NC-ND) licence. This licence only allows you to download this work and share it with others as long as you credit the authors, but you can’t change the article in any way or use it commercially. More information and the full terms of the licence here: https://creativecommons.org/licenses/ Takedown If you consider content in White Rose Research Online to be in breach of UK law, please notify us by emailing [email protected] including the URL of the record and the reason for the withdrawal request. [email protected] https://eprints.whiterose.ac.uk/ 1 Fundamentals of the Gut for Capsule Engineers 2 Lavinia Barducci*, Joseph C. Norton, Sunandita Sarker, Sayeed Mohammed, Ryan Jones, Pietro 3 Valdastri, Benjamin S. Terry 4 5 Lavinia Barducci*, M.Sc in Robotics and Automation Engineering, STORM Lab UK, Institute 6 of Robotics, School of Electronic and Electrical Engineering, University of Leeds, Woodhouse 7 Lane, Leeds, UK. -
The Function of Gastrointestinal Hormones in Obesity—Implications for the Regulation of Energy Intake
nutrients Review The Function of Gastrointestinal Hormones in Obesity—Implications for the Regulation of Energy Intake Mona Farhadipour and Inge Depoortere * Translational Research in Gastrointestinal Disorders, Gut Peptide Research Lab, University of Leuven, Gasthuisberg, 3000 Leuven, Belgium; [email protected] * Correspondence: [email protected] Abstract: The global burden of obesity and the challenges of prevention prompted researchers to investigate the mechanisms that control food intake. Food ingestion triggers several physiological responses in the digestive system, including the release of gastrointestinal hormones from enteroen- docrine cells that are involved in appetite signalling. Disturbed regulation of gut hormone release may affect energy homeostasis and contribute to obesity. In this review, we summarize the changes that occur in the gut hormone balance during the pre- and postprandial state in obesity and the alterations in the diurnal dynamics of their plasma levels. We further discuss how obesity may affect nutrient sensors on enteroendocrine cells that sense the luminal content and provoke alterations in their secretory profile. Gastric bypass surgery elicits one of the most favorable metabolic outcomes in obese patients. We summarize the effect of different strategies to induce weight loss on gut enteroendocrine function. Although the mechanisms underlying obesity are not fully understood, restoring the gut hormone balance in obesity by targeting nutrient sensors or by combination therapy Citation: Farhadipour, M.; with gut peptide mimetics represents a novel strategy to ameliorate obesity. Depoortere, I. The Function of Gastrointestinal Hormones in Keywords: obesity; gastrointestinal hormones; nutrient sensing; circadian clock; gastric bypass surgery Obesity—Implications for the Regulation of Energy Intake. Nutrients 2021, 13, 1839. -
The Interdigestive Motor Complex of Normal Subjects and Patients with Bacterial Overgrowth of the Small Intestine
The Interdigestive Motor Complex of Normal Subjects and Patients with Bacterial Overgrowth of the Small Intestine G. Vantrappen, … , J. Hellemans, Y. Ghoos J Clin Invest. 1977;59(6):1158-1166. https://doi.org/10.1172/JCI108740. Research Article Intraluminal pressures were measured in the gastric antrum and at different levels of the upper small intestine in 18 normal subjects to investigate whether or not the interdigestive motor complex, identified in several animal species, occurs in man and, if so, to determine its characteristics. In all normal subjects, the activity front of the interdigestive motor complex was readily identified as an uninterrupted burst of rhythmic contraction waves that progressed down the intestine and that was followed by a period of quiescence. Quantitative analysis of various parameters of the complex and simultaneous radiological and manometrical observations revealed that it resembled closely the canine interdigestive motor complex. To test the hypothesis that disorders of this motor complex may lead to bacterial overgrowth in the small 14 intestine, similar studies were performed in 18 patients with a positive CO2 bile acid breath test and in an additional 14 control group of 9 patients with a normal CO2 breath test. All but five patients had normal interdigestive motor complexes. The five patients in whom the motor complex was absent or greatly disordered had bacterial overgrowth as 14 evidenced by CO2 bile acid breath tests before and after antibiotics. These studies establish the presence and define the characteristics of the normal interdigestive motor complex in man. They also suggest that bacterial overgrowth may be due to a […] Find the latest version: https://jci.me/108740/pdf The Interdigestive Motor Complex of Normal Subjects and Patients with Bacterial Overgrowth of the Small Intestine G. -
Gastroparesis: Introduction
Gastroparesis: Introduction Gastroparesis, or gastric stasis , is a disorder of delayed gastric emptying in the absence of mechanical obstruction. It is manifest clinically through a set of largely non-specific symptoms such as early satiety , bloating, nausea, anorexia , vomiting, abdominal pain, and weight loss. Among these, vomiting and post-prandial fullness are the most specific. Common causes include diabetes mellitus , prior gastric surgery with or without vagotomy , a preceding infectious illness, pseudo-obstruction, collagen vascular disorders, and anorexia nervosa. Gastroparesis often presents as a subclinical disorder; hence there is no true estimate of its incidence or prevalence. However, it has been reported that between 30-50% of diabetics suffer from delayed gastric emptying. The prevalence of suggestive symptoms (e.g. nausea, vomiting) is much lower—with only about 10% of diabetics affected. Figure 1. Location of the stomach in the body Gastric Motor Physiology Normal gastric motility/emptying requires an integrated, coordinated interplay between the sympathetic, parasympathetic, and intrinsic-gut (enteric) nervous systems, and the gastrointestinal smooth muscle cells. Disturbance at any level has the potential to alter gastric function, and ultimately affect gastric emptying. To better understand gastric motility, it is important to be familiar with both the functional zones and the major digestive functions of the stomach —including the difference between an empty and a full stomach. On a functional basis, the stomach may be subdivided into two regions: 1. The proximal stomach comprises the cardia, fundus, and body—and is characterized by a thin layer of muscle that produces relatively weak contractions. Upon the ingestion of food, the proximal stomach exhibits receptive relaxation, with very little increase in intragastric pressure.