A Newly Identified Rab-Gdi Paralogue Has a Role in Neural Development in Amphibia
Total Page:16
File Type:pdf, Size:1020Kb
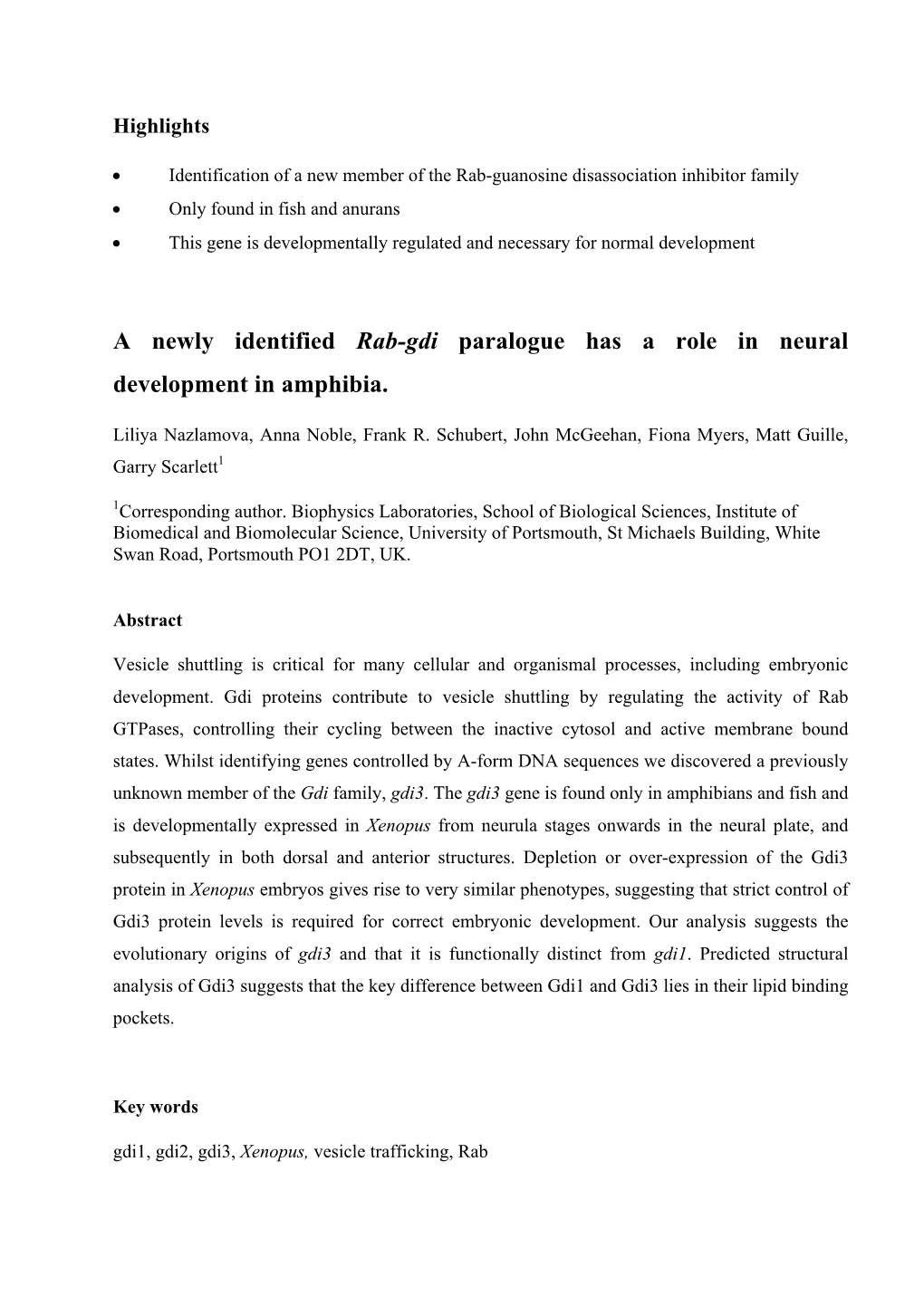
Load more
Recommended publications
-
Snps) Distant from Xenobiotic Response Elements Can Modulate Aryl Hydrocarbon Receptor Function: SNP-Dependent CYP1A1 Induction S
Supplemental material to this article can be found at: http://dmd.aspetjournals.org/content/suppl/2018/07/06/dmd.118.082164.DC1 1521-009X/46/9/1372–1381$35.00 https://doi.org/10.1124/dmd.118.082164 DRUG METABOLISM AND DISPOSITION Drug Metab Dispos 46:1372–1381, September 2018 Copyright ª 2018 by The American Society for Pharmacology and Experimental Therapeutics Single Nucleotide Polymorphisms (SNPs) Distant from Xenobiotic Response Elements Can Modulate Aryl Hydrocarbon Receptor Function: SNP-Dependent CYP1A1 Induction s Duan Liu, Sisi Qin, Balmiki Ray,1 Krishna R. Kalari, Liewei Wang, and Richard M. Weinshilboum Division of Clinical Pharmacology, Department of Molecular Pharmacology and Experimental Therapeutics (D.L., S.Q., B.R., L.W., R.M.W.) and Division of Biomedical Statistics and Informatics, Department of Health Sciences Research (K.R.K.), Mayo Clinic, Rochester, Minnesota Received April 22, 2018; accepted June 28, 2018 ABSTRACT Downloaded from CYP1A1 expression can be upregulated by the ligand-activated aryl fashion. LCLs with the AA genotype displayed significantly higher hydrocarbon receptor (AHR). Based on prior observations with AHR-XRE binding and CYP1A1 mRNA expression after 3MC estrogen receptors and estrogen response elements, we tested treatment than did those with the GG genotype. Electrophoretic the hypothesis that single-nucleotide polymorphisms (SNPs) map- mobility shift assay (EMSA) showed that oligonucleotides with the ping hundreds of base pairs (bp) from xenobiotic response elements AA genotype displayed higher LCL nuclear extract binding after (XREs) might influence AHR binding and subsequent gene expres- 3MC treatment than did those with the GG genotype, and mass dmd.aspetjournals.org sion. -
Analysis of the Dynamics of Limb Transcriptomes During Mouse Development Istvan Gyurján1, Bernhard Sonderegger1, Felix Naef1,2 and Denis Duboule1,3*
Gyurján et al. BMC Developmental Biology 2011, 11:47 http://www.biomedcentral.com/1471-213X/11/47 RESEARCH ARTICLE Open Access Analysis of the dynamics of limb transcriptomes during mouse development Istvan Gyurján1, Bernhard Sonderegger1, Felix Naef1,2 and Denis Duboule1,3* Abstract Background: The development of vertebrate limbs has been a traditional system to study fundamental processes at work during ontogenesis, such as the establishment of spatial cellular coordinates, the effect of diffusible morphogenetic molecules or the translation between gene activity and morphogenesis. In addition, limbs are amongst the first targets of malformations in human and they display a huge realm of evolutionary variations within tetrapods, which make them a paradigm to study the regulatory genome. Results: As a reference resource for future biochemical and genetic analyses, we used genome-wide tiling arrays to establish the transcriptomes of mouse limb buds at three different stages, during which major developmental events take place. We compare the three time-points and discuss some aspects of these datasets, for instance related to transcriptome dynamics or to the potential association between active genes and the distribution of intergenic transcriptional activity. Conclusions: These datasets provide a valuable resource, either for research projects involving gene expression and regulation in developing mouse limbs, or as examples of tissue-specific, genome-wide transcriptional activities. Background regulators of the dorso-ventral (DV) patterning are Limb development has fascinated biologists for a cen- Wnt7a, expressed in dorsal ectoderm, and Engrailed,in tury, mostly because of the importance of these struc- ventral ectoderm, as well as Lmxb1,agenetranscribed tures in the evolution of land vertebrates and due to in dorsal mesenchyme. -
Aneuploidy: Using Genetic Instability to Preserve a Haploid Genome?
Health Science Campus FINAL APPROVAL OF DISSERTATION Doctor of Philosophy in Biomedical Science (Cancer Biology) Aneuploidy: Using genetic instability to preserve a haploid genome? Submitted by: Ramona Ramdath In partial fulfillment of the requirements for the degree of Doctor of Philosophy in Biomedical Science Examination Committee Signature/Date Major Advisor: David Allison, M.D., Ph.D. Academic James Trempe, Ph.D. Advisory Committee: David Giovanucci, Ph.D. Randall Ruch, Ph.D. Ronald Mellgren, Ph.D. Senior Associate Dean College of Graduate Studies Michael S. Bisesi, Ph.D. Date of Defense: April 10, 2009 Aneuploidy: Using genetic instability to preserve a haploid genome? Ramona Ramdath University of Toledo, Health Science Campus 2009 Dedication I dedicate this dissertation to my grandfather who died of lung cancer two years ago, but who always instilled in us the value and importance of education. And to my mom and sister, both of whom have been pillars of support and stimulating conversations. To my sister, Rehanna, especially- I hope this inspires you to achieve all that you want to in life, academically and otherwise. ii Acknowledgements As we go through these academic journeys, there are so many along the way that make an impact not only on our work, but on our lives as well, and I would like to say a heartfelt thank you to all of those people: My Committee members- Dr. James Trempe, Dr. David Giovanucchi, Dr. Ronald Mellgren and Dr. Randall Ruch for their guidance, suggestions, support and confidence in me. My major advisor- Dr. David Allison, for his constructive criticism and positive reinforcement. -
430.Full.Pdf
430 LETTER TO JMG J Med Genet: first published as 10.1136/jmg.39.6.430 on 1 June 2002. Downloaded from A novel 2 bp deletion in the TM4SF2 gene is associated with MRX58 F E Abidi, E Holinski-Feder, O Rittinger, F Kooy, H A Lubs, R E Stevenson, C E Schwartz ............................................................................................................................. J Med Genet 2002;39:430–433 linked mental retardation (XLMR) represents around 5% detection of any alteration at the splice junction. Since the of all MR, with a prevalence of 1 in 600 males.12Fifteen PCR products for exons 2 and 5 were greater than 310 bp, they to twenty percent of the total XLMR is the result of the were digested with HaeIII and PvuII respectively (table 1). X 3 fragile X syndrome. Non-fragile X mental retardation was Amplification was done in a PTC-200 thermocycler (MJ subdivided into syndromal and non-syndromal conditions by Research). Following PCR, 10 µl of the IPS loading dye (95% Neri et al4 in 1991. The syndromal XLMR entities (MRXS) are formamide, 10 mmol/l NaOH, 0.25% bromophenol blue, 0.25% those in which there is a specific pattern of physical, xylene cyanol) was added. The samples were denatured at neurological, or metabolic abnormalities associated with the 96°C for three minutes and loaded on a 0.5 × MDE gel (FMC) presence of mental retardation.5 Non-syndromic XLMR prepared in 0.6 × TBE. The gel was run at 8 W for 15-20 hours (MRX) are conditions in which a gene mutation causes men- at room temperature. -
Gene Ontology Functional Annotations and Pleiotropy
Network based analysis of genetic disease associations Sarah Gilman Submitted in partial fulfillment of the requirements for the degree of Doctor of Philosophy under the Executive Committee of the Graduate School of Arts and Sciences COLUMBIA UNIVERSITY 2014 © 2013 Sarah Gilman All Rights Reserved ABSTRACT Network based analysis of genetic disease associations Sarah Gilman Despite extensive efforts and many promising early findings, genome-wide association studies have explained only a small fraction of the genetic factors contributing to common human diseases. There are many theories about where this “missing heritability” might lie, but increasingly the prevailing view is that common variants, the target of GWAS, are not solely responsible for susceptibility to common diseases and a substantial portion of human disease risk will be found among rare variants. Relatively new, such variants have not been subject to purifying selection, and therefore may be particularly pertinent for neuropsychiatric disorders and other diseases with greatly reduced fecundity. Recently, several researchers have made great progress towards uncovering the genetics behind autism and schizophrenia. By sequencing families, they have found hundreds of de novo variants occurring only in affected individuals, both large structural copy number variants and single nucleotide variants. Despite studying large cohorts there has been little recurrence among the genes implicated suggesting that many hundreds of genes may underlie these complex phenotypes. The question -
Anti-GDI1 (Aa 399-447) Polyclonal Antibody (DPABH-15243) This Product Is for Research Use Only and Is Not Intended for Diagnostic Use
Anti-GDI1 (aa 399-447) polyclonal antibody (DPABH-15243) This product is for research use only and is not intended for diagnostic use. PRODUCT INFORMATION Antigen Description Regulates the GDP/GTP exchange reaction of most Rab proteins by inhibiting the dissociation of GDP from them, and the subsequent binding of GTP to them. Immunogen A region within a synthetic peptide: FCSCSYDATT HFETTCNDIK DIYKRMAGTA FDFENMKRKQ NDVFGEAEQ, corresponding to C terminal amino acids 399-447 of Human GDI1 Isotype IgG Source/Host Rabbit Species Reactivity Human Purification IgG fraction Conjugate Unconjugated Applications WB Format Liquid Size 100 μg Buffer Constituents: 2% Sucrose, PBS Preservative None Storage Shipped at 4°C. Upon delivery aliquot and store at -20°C or -80°C. Avoid repeated freeze / thaw cycles. GENE INFORMATION Gene Name GDI1 GDP dissociation inhibitor 1 [ Homo sapiens ] Official Symbol GDI1 Synonyms GDI1; GDP dissociation inhibitor 1; GDIL, MRX41, MRX48; rab GDP dissociation inhibitor alpha; FLJ41411; mental retardation; X linked 41; X linked 48; OPHN2; rab GDP dissociation inhibitor; 45-1 Ramsey Road, Shirley, NY 11967, USA Email: [email protected] Tel: 1-631-624-4882 Fax: 1-631-938-8221 1 © Creative Diagnostics All Rights Reserved alpha; RABGDIA; XAP 4; GDI-1; protein XAP-4; rab GDI alpha; oligophrenin-2; mental retardation, X-linked 48; rab GDP-dissociation inhibitor, alpha; guanosine diphosphate dissociation inhibitor 1; 1A; GDIL; MRX41; MRX48; XAP-4; RABGD1A; Entrez Gene ID 2664 Protein Refseq NP_001484 UniProt ID P31150 Chromosome Location Xq28 Pathway Rho GTPase cycle; Signal Transduction; Signaling by Rho GTPases; Signaling mediated by p38-alpha and p38-beta; Function GDP-dissociation inhibitor activity; GTPase activator activity; Rab GDP-dissociation inhibitor activity; protein binding; 45-1 Ramsey Road, Shirley, NY 11967, USA Email: [email protected] Tel: 1-631-624-4882 Fax: 1-631-938-8221 2 © Creative Diagnostics All Rights Reserved. -
Multidimensional Informatic Deconvolution Defines Gender
Mechanisms of Ageing and Development 184 (2019) 111150 Contents lists available at ScienceDirect Mechanisms of Ageing and Development journal homepage: www.elsevier.com/locate/mechagedev Multidimensional informatic deconvolution defines gender-specific roles of hypothalamic GIT2 in aging trajectories T Jaana van Gastela, Huan Caib, Wei-Na Congb, Wayne Chadwickc, Caitlin Daimonb, Hanne Leysena, Jhana O. Hendrickxa, Robin De Scheppera, Laura Vangenechtena, Jens Van Turnhouta, Jasper Verswyvela, Kevin G. Beckerd, Yongqing Zhangd, Elin Lehrmannd, William H. Wood IIId, Bronwen Martinb,e,**,1, Stuart Maudsleya,c,*,1 a Receptor Biology Lab, Department of Biomedical Sciences, University of Antwerp, 2610, Wilrijk, Belgium b Metabolism Unit, Laboratory of Clinical Investigation, National Institute on Aging, National Institutes of Health, Biomedical Research Center, 251 Bayview Boulevard, Baltimore, MD, 21224, United States c Receptor Pharmacology Unit, Laboratory of Neuroscience, National Institute on Aging, National Institutes of Health, Biomedical Research Center, 251 Bayview Boulevard, Baltimore, MD, 21224, United States d Gene Expression and Genomics Unit, Laboratory of Genetics and Genomics, National Institute on Aging, National Institutes of Health, Biomedical Research Center, 251 Bayview Boulevard, Baltimore, MD, 21224, United States e Faculty of Pharmacy, Biomedical and Veterinary Sciences, University of Antwerp, 2610, Wilrijk, Belgium ARTICLE INFO ABSTRACT Keywords: In most species, females live longer than males. An understanding of this female longevity advantage will likely GIT2 uncover novel anti-aging therapeutic targets. Here we investigated the transcriptomic responses in the hy- Longevity pothalamus – a key organ for somatic aging control – to the introduction of a simple aging-related molecular Aging perturbation, i.e. GIT2 heterozygosity. Our previous work has demonstrated that GIT2 acts as a network con- Female troller of aging. -
Deciphering the Molecular Profile of Plaques, Memory Decline And
ORIGINAL RESEARCH ARTICLE published: 16 April 2014 AGING NEUROSCIENCE doi: 10.3389/fnagi.2014.00075 Deciphering the molecular profile of plaques, memory decline and neuron loss in two mouse models for Alzheimer’s disease by deep sequencing Yvonne Bouter 1†,Tim Kacprowski 2,3†, Robert Weissmann4, Katharina Dietrich1, Henning Borgers 1, Andreas Brauß1, Christian Sperling 4, Oliver Wirths 1, Mario Albrecht 2,5, Lars R. Jensen4, Andreas W. Kuss 4* andThomas A. Bayer 1* 1 Division of Molecular Psychiatry, Georg-August-University Goettingen, University Medicine Goettingen, Goettingen, Germany 2 Department of Bioinformatics, Institute of Biometrics and Medical Informatics, University Medicine Greifswald, Greifswald, Germany 3 Department of Functional Genomics, Interfaculty Institute for Genetics and Functional Genomics, University Medicine Greifswald, Greifswald, Germany 4 Human Molecular Genetics, Department for Human Genetics of the Institute for Genetics and Functional Genomics, Institute for Human Genetics, University Medicine Greifswald, Ernst-Moritz-Arndt University Greifswald, Greifswald, Germany 5 Institute for Knowledge Discovery, Graz University of Technology, Graz, Austria Edited by: One of the central research questions on the etiology of Alzheimer’s disease (AD) is the Isidro Ferrer, University of Barcelona, elucidation of the molecular signatures triggered by the amyloid cascade of pathological Spain events. Next-generation sequencing allows the identification of genes involved in disease Reviewed by: Isidro Ferrer, University of Barcelona, processes in an unbiased manner. We have combined this technique with the analysis of Spain two AD mouse models: (1) The 5XFAD model develops early plaque formation, intraneu- Dietmar R. Thal, University of Ulm, ronal Ab aggregation, neuron loss, and behavioral deficits. (2)TheTg4–42 model expresses Germany N-truncated Ab4–42 and develops neuron loss and behavioral deficits albeit without plaque *Correspondence: formation. -
University of California, San Diego
UC San Diego UC San Diego Electronic Theses and Dissertations Title The post-terminal differentiation fate of RNAs revealed by next-generation sequencing Permalink https://escholarship.org/uc/item/7324r1rj Author Lefkowitz, Gloria Kuo Publication Date 2012 Peer reviewed|Thesis/dissertation eScholarship.org Powered by the California Digital Library University of California UNIVERSITY OF CALIFORNIA, SAN DIEGO The post-terminal differentiation fate of RNAs revealed by next-generation sequencing A dissertation submitted in partial satisfaction of the requirements for the degree Doctor of Philosophy in Biomedical Sciences by Gloria Kuo Lefkowitz Committee in Charge: Professor Benjamin D. Yu, Chair Professor Richard Gallo Professor Bruce A. Hamilton Professor Miles F. Wilkinson Professor Eugene Yeo 2012 Copyright Gloria Kuo Lefkowitz, 2012 All rights reserved. The Dissertation of Gloria Kuo Lefkowitz is approved, and it is acceptable in quality and form for publication on microfilm and electronically: __________________________________________________________________ __________________________________________________________________ __________________________________________________________________ __________________________________________________________________ __________________________________________________________________ Chair University of California, San Diego 2012 iii DEDICATION Ma and Ba, for your early indulgence and support. Matt and James, for choosing more practical callings. Roy, my love, for patiently sharing the ups and downs -
The Gene Expression Profile Represents the Molecular Nature of Liver Metastasis in Colorectal Cancer
129-138 6/12/06 19:32 Page 129 INTERNATIONAL JOURNAL OF ONCOLOGY 30: 129-138, 2007 129 The gene expression profile represents the molecular nature of liver metastasis in colorectal cancer MAKOTO YAMASAKI1,2, ICHIRO TAKEMASA1, TAKAMICHI KOMORI1,2, SHOUJI WATANABE2, MITSUGU SEKIMOTO1, YUICHIRO DOKI1, KENICHI MATSUBARA2 and MORITO MONDEN1 1Department of Surgery and Clinical Oncology, Graduate School of Medicine, Osaka University, 2-2 Yamadaoka, Suita, Osaka 565-0879; 2DNA Chip Research Inc., 1-1-43 Suehiro, Tsurumi, Yokohama, Kanagawa 230-0045, Japan Received March 13, 2006; Accepted August 23, 2006 Abstract. The major cause of death in colorectal cancer is lesion rather than one of a non-metastasized primary tumor. related to liver metastasis. Although the metastatic process Using the supervised classification approach, the expression has been well studied, many aspects of the molecular genetic profile of these genes allowed the classification of tumors basis of metastasis remain unclear. Elucidation of the diagnosed as localized cancer into two classes, the localized molecular nature of liver metastasis is urgent to improve the and the metastasized class, according to their final metastatic outcome of colorectal cancer. We analyzed the chrono- status. The disease-free survival and overall survival were logical gene expression profiles of 104 colorectal samples significantly longer in the localized class than the metasta- corresponding to oncogenic development including normal sized class. Chronological analysis of the gene expression mucosa, localized and metastasized primary tumors, and liver profile provides a better understanding of the metastatic metastatic lesions as fundamental samples using a custom process. Our results suggest that the metastatic potential is cDNA microarray. -
An Analysis of the Ramosa1 Pathway in Zea Mays Utilizing CRISPR/Cas9 Knockouts
Iowa State University Capstones, Theses and Graduate Theses and Dissertations Dissertations 2019 An analysis of the ramosa1 pathway in Zea mays utilizing CRISPR/Cas9 knockouts Ryan James Arndorfer Iowa State University Follow this and additional works at: https://lib.dr.iastate.edu/etd Part of the Agriculture Commons, Genetics Commons, and the Plant Sciences Commons Recommended Citation Arndorfer, Ryan James, "An analysis of the ramosa1 pathway in Zea mays utilizing CRISPR/Cas9 knockouts" (2019). Graduate Theses and Dissertations. 17391. https://lib.dr.iastate.edu/etd/17391 This Thesis is brought to you for free and open access by the Iowa State University Capstones, Theses and Dissertations at Iowa State University Digital Repository. It has been accepted for inclusion in Graduate Theses and Dissertations by an authorized administrator of Iowa State University Digital Repository. For more information, please contact [email protected]. An analysis of the ramosa1 pathway in Zea mays utilizing CRISPR/Cas9 knockouts by Ryan Arndorfer A thesis submitted to the graduate faculty in partial fulfillment of the requirements for the degree of MASTER OF SCIENCE Major: Genetics and Genomics Program of Study Committee: Erik Vollbrecht, Major Professor Shuizhang Fei Philip Becraft The student author, whose presentation of the scholarship herein was approved by the program of study committee, is solely responsible for the content of this thesis. The Graduate College will ensure this thesis is globally accessible and will not permit alterations after a degree is conferred. Iowa State University Ames, Iowa 2019 Copyright © Ryan Arndorfer, 2019. All rights reserved. ii DEDICATION Lorem ipsum dolor sit amet, consectetur adipiscing elit, sed do eiusmod tempor incididunt ut labore et dolore magna aliqua. -
Datasheet: VPA00272 Product Details
Datasheet: VPA00272 Description: RABBIT ANTI GDI1 Specificity: GDI1 Format: Purified Product Type: PrecisionAb™ Polyclonal Isotype: Polyclonal IgG Quantity: 100 µl Product Details Applications This product has been reported to work in the following applications. This information is derived from testing within our laboratories, peer-reviewed publications or personal communications from the originators. Please refer to references indicated for further information. For general protocol recommendations, please visit www.bio-rad-antibodies.com/protocols. Yes No Not Determined Suggested Dilution Western Blotting 1/1000 PrecisionAb antibodies have been extensively validated for the western blot application. The antibody has been validated at the suggested dilution. Where this product has not been tested for use in a particular technique this does not necessarily exclude its use in such procedures. Further optimization may be required dependant on sample type. Target Species Human Product Form Purified IgG - liquid Preparation Rabbit polyclonal antibody purified by affinity chromatography Buffer Solution Phosphate buffered saline Preservative 0.09% Sodium Azide (NaN3) Stabilisers 1% Bovine Serum Albumin Immunogen KLH-conjugated synthetic peptide corresponding to aa 415-443 of human GDI1 External Database Links UniProt: P31150 Related reagents Entrez Gene: 2664 GDI1 Related reagents Synonyms GDIL, OPHN2, RABGDIA, XAP4 Specificity Rabbit anti Human GDI1 antibody recognizes GDI1, also known as guanosine diphosphate dissociation inhibitor 1, mental retardation, X-linked 48, oligophrenin-2, protein XAP-4, rab GDI alpha and rab GDP-dissociation inhibitor, alpha. Page 1 of 2 GDP dissociation inhibitors are proteins that regulate the GDP-GTP exchange reaction of members of the rab family, small GTP-binding proteins of the ras superfamily, that are involved in vesicular trafficking of molecules between cellular organelles.