Quantum Field Theory II
Total Page:16
File Type:pdf, Size:1020Kb
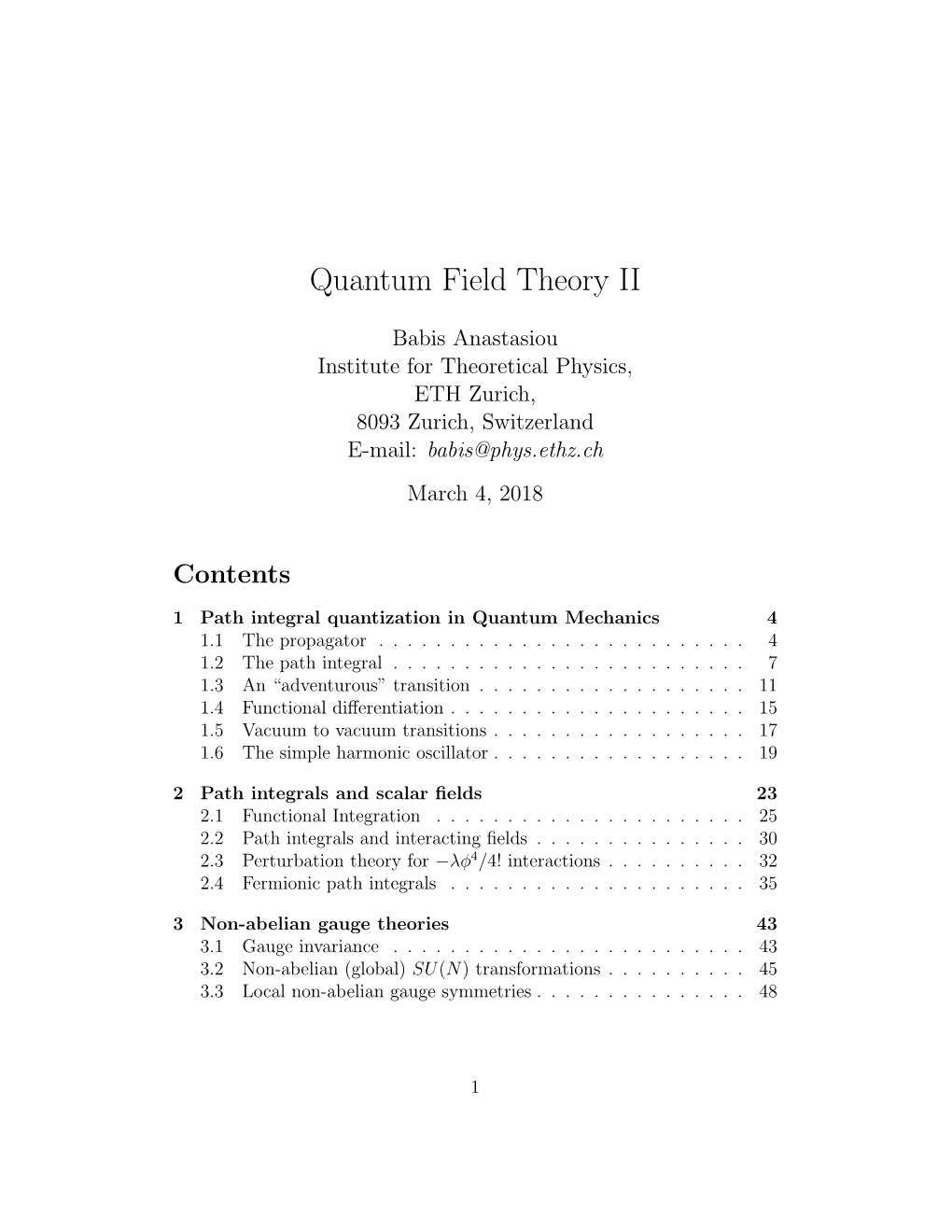
Load more
Recommended publications
-
Quantum Gravity and the Cosmological Constant Problem
Quantum Gravity and the Cosmological Constant Problem J. W. Moffat Perimeter Institute for Theoretical Physics, Waterloo, Ontario N2L 2Y5, Canada and Department of Physics and Astronomy, University of Waterloo, Waterloo, Ontario N2L 3G1, Canada October 15, 2018 Abstract A finite and unitary nonlocal formulation of quantum gravity is applied to the cosmological constant problem. The entire functions in momentum space at the graviton-standard model particle loop vertices generate an exponential suppression of the vacuum density and the cosmological constant to produce agreement with their observational bounds. 1 Introduction A nonlocal quantum field theory and quantum gravity theory has been formulated that leads to a finite, unitary and locally gauge invariant theory [1, 2, 3, 4, 5, 6, 7, 8, 9, 10, 11, 12, 13, 14]. For quantum gravity the finiteness of quantum loops avoids the problem of the non-renormalizabilty of local quantum gravity [15, 16]. The finiteness of the nonlocal quantum field theory draws from the fact that factors of exp[ (p2)/Λ2] are attached to propagators which suppress any ultraviolet divergences in Euclidean momentum space,K where Λ is an energy scale factor. An important feature of the field theory is that only the quantum loop graphs have nonlocal properties; the classical tree graph theory retains full causal and local behavior. Consider first the 4-dimensional spacetime to be approximately flat Minkowski spacetime. Let us denote by f a generic local field and write the standard local Lagrangian as [f]= [f]+ [f], (1) L LF LI where and denote the free part and the interaction part of the action, respectively, and LF LI arXiv:1407.2086v1 [gr-qc] 7 Jul 2014 1 [f]= f f . -
An Introduction to Quantum Field Theory
AN INTRODUCTION TO QUANTUM FIELD THEORY By Dr M Dasgupta University of Manchester Lecture presented at the School for Experimental High Energy Physics Students Somerville College, Oxford, September 2009 - 1 - - 2 - Contents 0 Prologue....................................................................................................... 5 1 Introduction ................................................................................................ 6 1.1 Lagrangian formalism in classical mechanics......................................... 6 1.2 Quantum mechanics................................................................................... 8 1.3 The Schrödinger picture........................................................................... 10 1.4 The Heisenberg picture............................................................................ 11 1.5 The quantum mechanical harmonic oscillator ..................................... 12 Problems .............................................................................................................. 13 2 Classical Field Theory............................................................................. 14 2.1 From N-point mechanics to field theory ............................................... 14 2.2 Relativistic field theory ............................................................................ 15 2.3 Action for a scalar field ............................................................................ 15 2.4 Plane wave solution to the Klein-Gordon equation ........................... -
Arxiv:Cond-Mat/0203258V1 [Cond-Mat.Str-El] 12 Mar 2002 AS 71.10.-W,71.27.+A PACS: Pnpolmi Oi Tt Hsc.Iscoeconnection Close Its High- of Physics
Large-N expansion based on the Hubbard-operator path integral representation and its application to the t J model − Adriana Foussats and Andr´es Greco Facultad de Ciencias Exactas Ingenier´ıa y Agrimensura and Instituto de F´ısica Rosario (UNR-CONICET). Av.Pellegrini 250-2000 Rosario-Argentina. (October 29, 2018) In the present work we have developed a large-N expansion for the t − J model based on the path integral formulation for Hubbard-operators. Our large-N expansion formulation contains diagram- matic rules, in which the propagators and vertex are written in term of Hubbard operators. Using our large-N formulation we have calculated, for J = 0, the renormalized O(1/N) boson propagator. We also have calculated the spin-spin and charge-charge correlation functions to leading order 1/N. We have compared our diagram technique and results with the existing ones in the literature. PACS: 71.10.-w,71.27.+a I. INTRODUCTION this constrained theory leads to the commutation rules of the Hubbard-operators. Next, by using path-integral The role of electronic correlations is an important and techniques, the correlation functional and effective La- open problem in solid state physics. Its close connection grangian were constructed. 1 with the phenomena of high-Tc superconductivity makes In Ref.[ 11], we found a particular family of constrained this problem relevant in present days. Lagrangians and showed that the corresponding path- One of the most popular models in the context of high- integral can be mapped to that of the slave-boson rep- 13,5 Tc superconductivity is the t J model. -
Effective Quantum Field Theories Thomas Mannel Theoretical Physics I (Particle Physics) University of Siegen, Siegen, Germany
Generating Functionals Functional Integration Renormalization Introduction to Effective Quantum Field Theories Thomas Mannel Theoretical Physics I (Particle Physics) University of Siegen, Siegen, Germany 2nd Autumn School on High Energy Physics and Quantum Field Theory Yerevan, Armenia, 6-10 October, 2014 T. Mannel, Siegen University Effective Quantum Field Theories: Lecture 1 Generating Functionals Functional Integration Renormalization Overview Lecture 1: Basics of Quantum Field Theory Generating Functionals Functional Integration Perturbation Theory Renormalization Lecture 2: Effective Field Thoeries Effective Actions Effective Lagrangians Identifying relevant degrees of freedom Renormalization and Renormalization Group T. Mannel, Siegen University Effective Quantum Field Theories: Lecture 1 Generating Functionals Functional Integration Renormalization Lecture 3: Examples @ work From Standard Model to Fermi Theory From QCD to Heavy Quark Effective Theory From QCD to Chiral Perturbation Theory From New Physics to the Standard Model Lecture 4: Limitations: When Effective Field Theories become ineffective Dispersion theory and effective field theory Bound Systems of Quarks and anomalous thresholds When quarks are needed in QCD É. T. Mannel, Siegen University Effective Quantum Field Theories: Lecture 1 Generating Functionals Functional Integration Renormalization Lecture 1: Basics of Quantum Field Theory Thomas Mannel Theoretische Physik I, Universität Siegen f q f et Yerevan, October 2014 T. Mannel, Siegen University Effective Quantum -
Macroscopic Quantum Phenomena in Interacting Bosonic Systems: Josephson flow in Liquid 4He and Multimode Schr¨Odingercat States
Macroscopic quantum phenomena in interacting bosonic systems: Josephson flow in liquid 4He and multimode Schr¨odingercat states by Tyler James Volkoff A dissertation submitted in partial satisfaction of the requirements for the degree of Doctor of Philosophy in Chemistry in the Graduate Division of the University of California, Berkeley Committee in charge: Professor K. Birgitta Whaley, Chair Professor Philip L. Geissler Professor Richard E. Packard Fall 2014 Macroscopic quantum phenomena in interacting bosonic systems: Josephson flow in liquid 4He and multimode Schr¨odingercat states Copyright 2014 by Tyler James Volkoff 1 Abstract Macroscopic quantum phenomena in interacting bosonic systems: Josephson flow in liquid 4He and multimode Schr¨odingercat states by Tyler James Volkoff Doctor of Philosophy in Chemistry University of California, Berkeley Professor K. Birgitta Whaley, Chair In this dissertation, I analyze certain problems in the following areas: 1) quantum dynam- ical phenomena in macroscopic systems of interacting, degenerate bosons (Parts II, III, and V), and 2) measures of macroscopicity for a large class of two-branch superposition states in separable Hilbert space (Part IV). Part I serves as an introduction to important concepts recurring in the later Parts. In Part II, a microscopic derivation of the effective action for the relative phase of driven, aperture-coupled reservoirs of weakly-interacting condensed bosons from a (3 + 1)D microscopic model with local U(1) gauge symmetry is presented. The effec- tive theory is applied to the transition from linear to sinusoidal current vs. phase behavior observed in recent experiments on liquid 4He driven through nanoaperture arrays. Part III discusses path-integral Monte Carlo (PIMC) numerical simulations of quantum hydrody- namic properties of reservoirs of He II communicating through simple nanoaperture arrays. -
The Mechanics of the Fermionic and Bosonic Fields: an Introduction to the Standard Model and Particle Physics
The Mechanics of the Fermionic and Bosonic Fields: An Introduction to the Standard Model and Particle Physics Evan McCarthy Phys. 460: Seminar in Physics, Spring 2014 Aug. 27,! 2014 1.Introduction 2.The Standard Model of Particle Physics 2.1.The Standard Model Lagrangian 2.2.Gauge Invariance 3.Mechanics of the Fermionic Field 3.1.Fermi-Dirac Statistics 3.2.Fermion Spinor Field 4.Mechanics of the Bosonic Field 4.1.Spin-Statistics Theorem 4.2.Bose Einstein Statistics !5.Conclusion ! 1. Introduction While Quantum Field Theory (QFT) is a remarkably successful tool of quantum particle physics, it is not used as a strictly predictive model. Rather, it is used as a framework within which predictive models - such as the Standard Model of particle physics (SM) - may operate. The overarching success of QFT lends it the ability to mathematically unify three of the four forces of nature, namely, the strong and weak nuclear forces, and electromagnetism. Recently substantiated further by the prediction and discovery of the Higgs boson, the SM has proven to be an extraordinarily proficient predictive model for all the subatomic particles and forces. The question remains, what is to be done with gravity - the fourth force of nature? Within the framework of QFT theoreticians have predicted the existence of yet another boson called the graviton. For this reason QFT has a very attractive allure, despite its limitations. According to !1 QFT the gravitational force is attributed to the interaction between two gravitons, however when applying the equations of General Relativity (GR) the force between two gravitons becomes infinite! Results like this are nonsensical and must be resolved for the theory to stand. -
Gauge-Fixing Degeneracies and Confinement in Non-Abelian Gauge Theories
PH YSICAL RKVIE% 0 VOLUME 17, NUMBER 4 15 FEBRUARY 1978 Gauge-fixing degeneracies and confinement in non-Abelian gauge theories Carl M. Bender Department of I'hysics, 8'ashington University, St. Louis, Missouri 63130 Tohru Eguchi Stanford Linear Accelerator Center, Stanford, California 94305 Heinz Pagels The Rockefeller University, New York, N. Y. 10021 (Received 28 September 1977) Following several suggestions of Gribov we have examined the problem of gauge-fixing degeneracies in non- Abelian gauge theories. First we modify the usual Faddeev-Popov prescription to take gauge-fixing degeneracies into account. We obtain a formal expression for the generating functional which is invariant under finite gauge transformations and which counts gauge-equivalent orbits only once. Next we examine the instantaneous Coulomb interaction in the canonical formalism with the Coulomb-gauge condition. We find that the spectrum of the Coulomb Green's function in an external monopole-hke field configuration has an accumulation of negative-energy bound states at E = 0. Using semiclassical methods we show that this accumulation phenomenon, which is closely linked with gauge-fixing degeneracies, modifies the usual Coulomb propagator from (k) ' to Iki ' for small )k[. This confinement behavior depends only on the long-range behavior of the field configuration. We thereby demonstrate the conjectured confinement property of non-Abelian gauge theories in the Coulomb gauge. I. INTRODUCTION lution to the theory. The failure of the Borel sum to define the theory and gauge-fixing degeneracy It has recently been observed by Gribov' that in are correlated phenomena. non-Abelian gauge theories, in contrast with Abe- In this article we examine the gauge-fixing de- lian theories, standard gauge-fixing conditions of generacy further. -
Large-Deviation Principles, Stochastic Effective Actions, Path Entropies
Large-deviation principles, stochastic effective actions, path entropies, and the structure and meaning of thermodynamic descriptions Eric Smith Santa Fe Institute, 1399 Hyde Park Road, Santa Fe, NM 87501, USA (Dated: February 18, 2011) The meaning of thermodynamic descriptions is found in large-deviations scaling [1, 2] of the prob- abilities for fluctuations of averaged quantities. The central function expressing large-deviations scaling is the entropy, which is the basis for both fluctuation theorems and for characterizing the thermodynamic interactions of systems. Freidlin-Wentzell theory [3] provides a quite general for- mulation of large-deviations scaling for non-equilibrium stochastic processes, through a remarkable representation in terms of a Hamiltonian dynamical system. A number of related methods now exist to construct the Freidlin-Wentzell Hamiltonian for many kinds of stochastic processes; one method due to Doi [4, 5] and Peliti [6, 7], appropriate to integer counting statistics, is widely used in reaction-diffusion theory. Using these tools together with a path-entropy method due to Jaynes [8], this review shows how to construct entropy functions that both express large-deviations scaling of fluctuations, and describe system-environment interactions, for discrete stochastic processes either at or away from equilibrium. A collection of variational methods familiar within quantum field theory, but less commonly applied to the Doi-Peliti construction, is used to define a “stochastic effective action”, which is the large-deviations rate function for arbitrary non-equilibrium paths. We show how common principles of entropy maximization, applied to different ensembles of states or of histories, lead to different entropy functions and different sets of thermodynamic state variables. -
Physics of Three-Dimensional Bosonic Topological Insulators: Surface-Deconfined Criticality and Quantized Magnetoelectric Effect
PHYSICAL REVIEW X 3, 011016 (2013) Physics of Three-Dimensional Bosonic Topological Insulators: Surface-Deconfined Criticality and Quantized Magnetoelectric Effect Ashvin Vishwanath Department of Physics, University of California, Berkeley, California 94720, USA Materials Science Division, Lawrence Berkeley National Laboratories, Berkeley, California 94720, USA T. Senthil Department of Physics, Massachusetts Institute of Technology, Cambridge, Massachusetts 02139, USA (Received 4 October 2012; revised manuscript received 31 December 2012; published 28 February 2013) We discuss physical properties of ‘‘integer’’ topological phases of bosons in D ¼ 3 þ 1 dimensions, protected by internal symmetries like time reversal and/or charge conservation. These phases invoke interactions in a fundamental way but do not possess topological order; they are bosonic analogs of free- fermion topological insulators and superconductors. While a formal cohomology-based classification of such states was recently discovered, their physical properties remain mysterious. Here, we develop a field-theoretic description of several of these states and show that they possess unusual surface states, which, if gapped, must either break the underlying symmetry or develop topological order. In the latter case, symmetries are implemented in a way that is forbidden in a strictly two-dimensional theory. While these phases are the usual fate of the surface states, exotic gapless states can also be realized. For example, tuning parameters can naturally lead to a deconfined quantum critical point or, in other situations, to a fully symmetric vortex metal phase. We discuss cases where the topological phases are characterized by a quantized magnetoelectric response , which, somewhat surprisingly, is an odd multiple of 2.Two different surface theories are shown to capture these phenomena: The first is a nonlinear sigma model with a topological term. -
Funaional Integration for Solving the Schroedinger Equation
Comitato Nazionale Energia Nucleare Funaional Integration for Solving the Schroedinger Equation A. BOVE. G. FANO. A.G. TEOLIS RT/FIMÀ(7$ Comitato Nazionale Energia Nucleare Funaional Integration for Solving the Schroedinger Equation A. BOVE, G.FANO, A.G.TEOLIS 3 1. INTRODUCTION Vesto pervenuto il 2 agosto 197) It ia well-known (aee e.g. tei. [lj - |3| ) that the infinite-diaeneional r«nctional integration ia a powerful tool in all the evolution processes toth of the type of the heat -tanefer or Scbroediager equation. Since the 'ieid of applications of functional integration ia rapidly increasing, it *«*ae dcairahle to study the «stood from the nuaerical point of view, at leaat in siaple caaea. Such a orograa baa been already carried out by Cria* and Scorer (ace ref. |«| - |7| ), via Monte-Carlo techniques. We use extensively an iteration aethod which coneiecs in successively squa£ ing < denaicy matrix (aee Eq.(9)), since in principle ic allows a great accuracy. This aetbod was used only tarely before (aee ref. |4| ). Fur- chersjore we give an catiaate of che error (e«e Eqs. (U),(26)) boch in Che caaa of Wiener and unleabeck-Ometein integral. Contrary to the cur rent opinion (aae ref. |13| ), we find that the last integral ia too sen sitive to the choice of the parameter appearing in the haraonic oscilla tor can, in order to be useful except in particular caaea. In this paper we study the one-particle spherically sysssetric case; an application to the three nucleon probUa is in progress (sea ref. |U| ). St»wp«wirìf^m<'oUN)p^woMG>miutoN«i»OMtop«fl'Ht>p>i«Wi*faif»,Piijl(Mi^fcri \ptt,mt><r\A, i uwh P.ei#*mià, Uffcio Eanfeai SaMfiM», " ~ Hot*, Vi.1. -
Gauge Symmetry Breaking in Gauge Theories---In Search of Clarification
Gauge Symmetry Breaking in Gauge Theories—In Search of Clarification Simon Friederich [email protected] Universit¨at Wuppertal, Fachbereich C – Mathematik und Naturwissenschaften, Gaußstr. 20, D-42119 Wuppertal, Germany Abstract: The paper investigates the spontaneous breaking of gauge sym- metries in gauge theories from a philosophical angle, taking into account the fact that the notion of a spontaneously broken local gauge symmetry, though widely employed in textbook expositions of the Higgs mechanism, is not supported by our leading theoretical frameworks of gauge quantum the- ories. In the context of lattice gauge theory, the statement that local gauge symmetry cannot be spontaneously broken can even be made rigorous in the form of Elitzur’s theorem. Nevertheless, gauge symmetry breaking does occur in gauge quantum field theories in the form of the breaking of remnant subgroups of the original local gauge group under which the theories typi- cally remain invariant after gauge fixing. The paper discusses the relation between these instances of symmetry breaking and phase transitions and draws some more general conclusions for the philosophical interpretation of gauge symmetries and their breaking. 1 Introduction The interpretation of symmetries and symmetry breaking has been recog- nized as a central topic in the philosophy of science in recent years. Gauge symmetries, in particular, have attracted a considerable amount of inter- arXiv:1107.4664v2 [physics.hist-ph] 5 Aug 2012 est due to the central role they play in our most successful theories of the fundamental constituents of nature. The standard model of elementary par- ticle physics, for instance, is formulated in terms of gauge symmetry, and so are its most discussed extensions. -
Finite Quantum Field Theory and Renormalization Group
Finite Quantum Field Theory and Renormalization Group M. A. Greena and J. W. Moffata;b aPerimeter Institute for Theoretical Physics, Waterloo, Ontario N2L 2Y5, Canada bDepartment of Physics and Astronomy, University of Waterloo, Waterloo, Ontario N2L 3G1, Canada September 15, 2021 Abstract Renormalization group methods are applied to a scalar field within a finite, nonlocal quantum field theory formulated perturbatively in Euclidean momentum space. It is demonstrated that the triviality problem in scalar field theory, the Higgs boson mass hierarchy problem and the stability of the vacuum do not arise as issues in the theory. The scalar Higgs field has no Landau pole. 1 Introduction An alternative version of the Standard Model (SM), constructed using an ultraviolet finite quantum field theory with nonlocal field operators, was investigated in previous work [1]. In place of Dirac delta-functions, δ(x), the theory uses distributions (x) based on finite width Gaussians. The Poincar´eand gauge invariant model adapts perturbative quantumE field theory (QFT), with a finite renormalization, to yield finite quantum loops. For the weak interactions, SU(2) U(1) is treated as an ab initio broken symmetry group with non- zero masses for the W and Z intermediate× vector bosons and for left and right quarks and leptons. The model guarantees the stability of the vacuum. Two energy scales, ΛM and ΛH , were introduced; the rate of asymptotic vanishing of all coupling strengths at vertices not involving the Higgs boson is controlled by ΛM , while ΛH controls the vanishing of couplings to the Higgs. Experimental tests of the model, using future linear or circular colliders, were proposed.