A Cubesat Centrifuge for Long Duration Milligravity Research
Total Page:16
File Type:pdf, Size:1020Kb
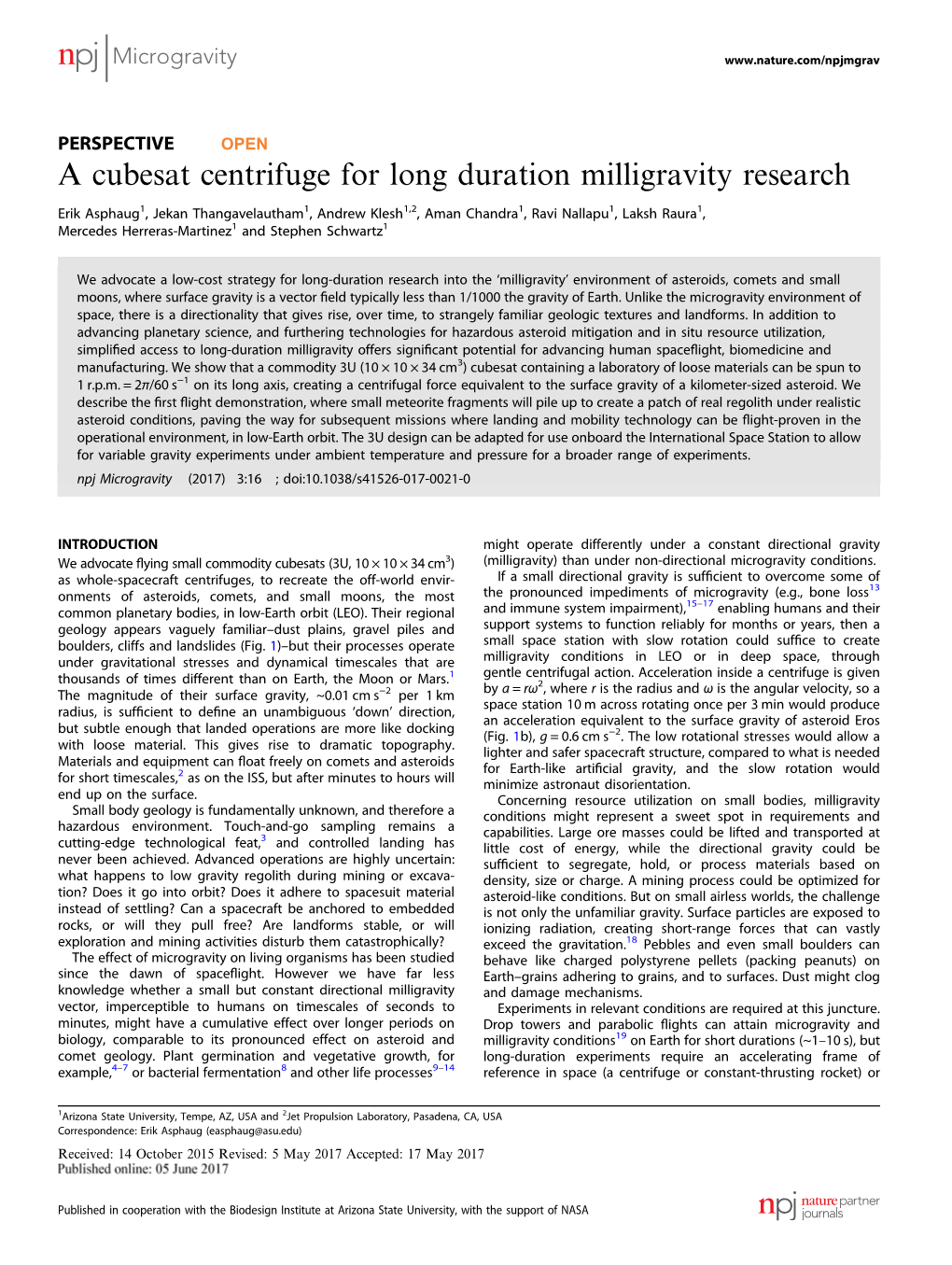
Load more
Recommended publications
-
Report Resumes
REPORT RESUMES ED 019 218 88 SE 004 494 A RESOURCE BOOK OF AEROSPACE ACTIVITIES, K-6. LINCOLN PUBLIC SCHOOLS, NEBR. PUB DATE 67 EDRS PRICEMF.41.00 HC-S10.48 260P. DESCRIPTORS- *ELEMENTARY SCHOOL SCIENCE, *PHYSICAL SCIENCES, *TEACHING GUIDES, *SECONDARY SCHOOL SCIENCE, *SCIENCE ACTIVITIES, ASTRONOMY, BIOGRAPHIES, BIBLIOGRAPHIES, FILMS, FILMSTRIPS, FIELD TRIPS, SCIENCE HISTORY, VOCABULARY, THIS RESOURCE BOOK OF ACTIVITIES WAS WRITTEN FOR TEACHERS OF GRADES K-6, TO HELP THEM INTEGRATE AEROSPACE SCIENCE WITH THE REGULAR LEARNING EXPERIENCES OF THE CLASSROOM. SUGGESTIONS ARE MADE FOR INTRODUCING AEROSPACE CONCEPTS INTO THE VARIOUS SUBJECT FIELDS SUCH AS LANGUAGE ARTS, MATHEMATICS, PHYSICAL EDUCATION, SOCIAL STUDIES, AND OTHERS. SUBJECT CATEGORIES ARE (1) DEVELOPMENT OF FLIGHT, (2) PIONEERS OF THE AIR (BIOGRAPHY),(3) ARTIFICIAL SATELLITES AND SPACE PROBES,(4) MANNED SPACE FLIGHT,(5) THE VASTNESS OF SPACE, AND (6) FUTURE SPACE VENTURES. SUGGESTIONS ARE MADE THROUGHOUT FOR USING THE MATERIAL AND THEMES FOR DEVELOPING INTEREST IN THE REGULAR LEARNING EXPERIENCES BY INVOLVING STUDENTS IN AEROSPACE ACTIVITIES. INCLUDED ARE LISTS OF SOURCES OF INFORMATION SUCH AS (1) BOOKS,(2) PAMPHLETS, (3) FILMS,(4) FILMSTRIPS,(5) MAGAZINE ARTICLES,(6) CHARTS, AND (7) MODELS. GRADE LEVEL APPROPRIATENESS OF THESE MATERIALSIS INDICATED. (DH) 4:14.1,-) 1783 1490 ,r- 6e tt*.___.Vhf 1842 1869 LINCOLN PUBLICSCHOOLS A RESOURCEBOOK OF AEROSPACEACTIVITIES U.S. DEPARTMENT OF HEALTH, EDUCATION & WELFARE OFFICE OF EDUCATION K-6) THIS DOCUMENT HAS BEEN REPRODUCED EXACTLY AS RECEIVED FROM THE PERSON OR ORGANIZATION ORIGINATING IT.POINTS OF VIEW OR OPINIONS STATED DO NOT NECESSARILY REPRESENT OFFICIAL OFFICE OF EDUCATION POSITION OR POLICY. 1919 O O Vj A PROJECT FUNDED UNDER TITLE HIELEMENTARY AND SECONDARY EDUCATION ACT A RESOURCE BOOK OF AEROSPACE ACTIVITIES (K-6) The work presentedor reported herein was performed pursuant to a Grant from the U. -
Up, Up, and Away by James J
www.astrosociety.org/uitc No. 34 - Spring 1996 © 1996, Astronomical Society of the Pacific, 390 Ashton Avenue, San Francisco, CA 94112. Up, Up, and Away by James J. Secosky, Bloomfield Central School and George Musser, Astronomical Society of the Pacific Want to take a tour of space? Then just flip around the channels on cable TV. Weather Channel forecasts, CNN newscasts, ESPN sportscasts: They all depend on satellites in Earth orbit. Or call your friends on Mauritius, Madagascar, or Maui: A satellite will relay your voice. Worried about the ozone hole over Antarctica or mass graves in Bosnia? Orbital outposts are keeping watch. The challenge these days is finding something that doesn't involve satellites in one way or other. And satellites are just one perk of the Space Age. Farther afield, robotic space probes have examined all the planets except Pluto, leading to a revolution in the Earth sciences -- from studies of plate tectonics to models of global warming -- now that scientists can compare our world to its planetary siblings. Over 300 people from 26 countries have gone into space, including the 24 astronauts who went on or near the Moon. Who knows how many will go in the next hundred years? In short, space travel has become a part of our lives. But what goes on behind the scenes? It turns out that satellites and spaceships depend on some of the most basic concepts of physics. So space travel isn't just fun to think about; it is a firm grounding in many of the principles that govern our world and our universe. -
Surface Gravity and Interstellar Settlement (Version 3.0 September 2016)
Surface Gravity and Interstellar Settlement (version 3.0 September 2016) by Gerald D. Nordley 1238 Prescott Avenue Sunnyvale, CA 94089-2334 [email protected] Abstract. Gravity determines the scale of many physical Jupiter's inner satellites melts the ice of Europa and drives phenomena on and above a world's surface. Assuming that tool- volcanoes on Io, and that Mars in the past has had rain and using intelligences come from, and would settle, worlds with solid floods serves notice that worlds that are significantly less surfaces and gravities equal or less than that of their origin, we massive than Earth and have significantly less surface gravity note that such worlds in the Solar System have surface gravities can still have the deep atmospheres and liquid water significantly lower than that of Earth. The distribution of these temperatures needed to sustain life. surface gravities clumps around factors of about 2.5 and favors worlds with about one-sixth Earth gravity, conventionally 2. Surface Gravity Basics considered too small to retain atmospheres. Titan, however, Mass is, roughly, a measure of the amount of substance. shows that low surface gravity does not, in itself, preclude the Weight is the force by which that substance is attracted to presence a substantial atmosphere. Where small worlds have another mass. This force is directly proportional to the product of low exobase temperatures,and protection from stellar winds, both masses and inversely proportional to the square of the substantial atmospheres may be retained. distance between them. A spherically symmetric planet can be Significantly lower surface gravity affects a number of physical treated as if all the mass were at the center, so the relevant phenomena that affect environment and technological evolution-- distance is the radius from the center to the object. -
Appendix a Apollo 15: “The Problem We Brought Back from the Moon”
Appendix A Apollo 15: “The Problem We Brought Back From the Moon” Postal Covers Carried on Apollo 151 Among the best known collectables from the Apollo Era are the covers flown onboard the Apollo 15 mission in 1971, mainly because of what the mission’s Lunar Module Pilot, Jim Irwin, called “the problem we brought back from the Moon.” [1] The crew of Apollo 15 carried out one of the most complete scientific explorations of the Moon and accomplished several firsts, including the first lunar roving vehicle that was operated on the Moon to extend the range of exploration. Some 81 kilograms (180 pounds) of lunar surface samples were returned for anal- ysis, and a battery of very productive lunar surface and orbital experiments were conducted, including the first EVA in deep space. [2] Yet the Apollo 15 crew are best remembered for carrying envelopes to the Moon, and the mission is remem- bered for the “great postal caper.” [3] As noted in Chapter 7, Apollo 15 was not the first mission to carry covers. Dozens were carried on each flight from Apollo 11 onwards (see Table 1 for the complete list) and, as Apollo 15 Commander Dave Scott recalled in his book, the whole business had probably been building since Mercury, through Gemini and into Apollo. [4] People had a fascination with objects that had been carried into space, and that became more and more popular – and valuable – as the programs progressed. Right from the start of the Mercury program, each astronaut had been allowed to carry a certain number of personal items onboard, with NASA’s permission, in 1 A first version of this material was issued as Apollo 15 Cover Scandal in Orbit No. -
Gemini 6 Press
NATIONAL AERONAUTICS AND SPACE ADMINISTRATION TELS. WO 2-4155 WASHINGTON, D.C. 20546 WO 3-6,925 FOR RELEASE: WEDNESDAY AM, S October 20, 1965 REUSE NO: 65-327 PROJECT: 6 CONTENTS Title Pa_e GENERAL NEWS RELEASE ........... '.......... 1-6 Orbits - Revolutions ...................... 7-10 Launch Vehicle Countdown .......... -....... ll-12 Experiments ............................... 13-I_ RadiationSynoptic Terrain...................Photography _ .................'.- 15,1b Synoptic Weather Photography ........... 17 Bio-Ch_mical Analysis of Body Fluids--- 17-18 70MMHasselblad Camera .................... 19 16MMMaurer Movie Camera .................. 20 Gemini 6 Nominal Maneuvers ................ 21 Checks while docked ....................... 22 Laser Beam0bservation .................... 23-24 Y_mmediate Preflight Crew Activities ....... 25-26 Mission Description ....................... 27-32 Manned Space Flight Tracking Network Gemini 6 Mission Requirements ........ 33 Tracking .................................. 34- 43 Network Configuration ..................... 44 Crew Safety ............................... _-47 Gemini Parachute Landing Sequence ......... 48 I AbortAtlanticProceduresRecovery..........................Area Communications ..... 49 Planned and Contingency Landing Areas ..... 50-51 Weather Requirements ...................... 52 Body Waste Disposal ....................... Gemini 6 Suit ............................. T FoodMedical.............Checks ............................"................ --- ..... 555_ WaherMen_s ...............Measuring -
Effect of Gravity on Current & Their Implications on Planets
Crimson Publishers Research Article Wings to the Research Effect of Gravity on Current & Their Implications on Planets Saifullah Khalid* IEEE, India Abstract ISSN: 2640-9739 any variations in the effect of gravity at the atomic level and macroscopic level. The effect of gravity has beenThis paper calculated presents for various the effect planets. of gravity Consequently, on the atomic suggestions level. Various have researchbeen made has for been the considered space equipment to find manufacturers to consider the variation in current density due to gravity for the planets. Keywords: Gravity; Current; Charge; Earth; Planets Introduction Anything that has mass has gravity too. Objects with greater mass have greater gravity. With distance also, gravity gets weaker. Therefore, the smaller the bodies are, the greater their gravitational force is [1-5]. The gravity of Earth originates from all of its mass. All its mass makes the whole mass in the body a combined gravitational pull on. If we are on a planet *1Corresponding author: Saifullah Khalid, Member, IEEE, India with less mass than Earth, we’d be weighing less than here. Gravity is a fundamental force of Nature, one we Earthlings prefer to take for granted. And you can’t blame us. Having evolved Submission: March 24, 2021 in Earth’s climate throughout billions of years, we are used to living with the tug of a steady Published: August 03, 2021 1g (or 9.8m/s2). Yet gravity is a very tenuous and precious thing for those who have gone into space or set foot on the Moon [6]. Isaac Newton and Albert Einstein’s works dominate the Volume 2 - Issue 2 development of the theory of gravity. -
PHYSICAL GEOLOGY (WITH LABORATORY) Syllabus EPS 3300 – PHYSICAL GEOLOGY (4 Crs
Kingsborough Community College The City University of New York Department of Physical Sciences EPS 3300 – PHYSICAL GEOLOGY (WITH LABORATORY) Syllabus EPS 3300 – PHYSICAL GEOLOGY (4 crs. 6 hrs.) Study of the nature of the Earth and its processes includes: mineral and rock classification, analysis of the agents of weathering and erosion, dynamics of the Earth’s crust as manifest in mountain building, volcanoes and earthquakes, recent data concerning the geology of other planets, field and laboratory techniques of the geologist. ----Prerequisites: Passed, exempt, or completed developmental course work for the CUNY Assessment Tests in Reading, Writing, and ACCUPLACER CUNY Assessment Test in Math or Department permission ----Required Core: Life and Physical Sciences----- Flexible Core: Scientific World (Group E) Section: SECTION NUMBER Time: LECTURE AND LABORATORY SCHEDULE FOR SECTION Room: ROOM (S) FOR SECTION Instructor: INSTRUCTOR FOR SECTION Email: EMAIL ADDRESS FOR INSTRUCTOR FOR SECTION Office Hours: OFFICE HOURS FOR INSTRUCTOR FOR SECTION Source materials: An Introduction to Physical Geology10th or 11th or latest edition, by Tarbuck, Lutgens & Tasa Student Learning Outcomes Students will: Differentiate between the three types of plate boundaries by noting common geologic features and processes. Classify common physical properties and differentiate minerals and rocks. Summarize the relationship between the chemical and physical properties of minerals. Analyze igneous, metamorphic, and sedimentary rocks to determine how they formed. Compare how different types of magma form and explain their relationship to the formation of intrusive and volcanic igneous features. Compare and contrast weathering among different rock types and different environments. Identify strata, faults, and folds in geologic sections and summarize the forces and tectonic settings that lead to their formation. -
Summary of Lesson
Energy Skate Park Name High School Student Activity Class Open the TI-Nspire document energy-skate-park.tns. In this simulation, you will observe the changing potential and kinetic energies of a skateboarder-celestial body system as she moves over a track with negligible friction. You will also explore the relationship between the system’s potential and kinetic energy, and how they affect the total energy of the system. Finally, you will explore the impact of friction on these potential and kinetic energies. Read the information on page 1.1. Move to page 1.2. To start the simulation, select and drag the skateboarder up to the top left side of the track. Then, release him. You will see the skateboarder moving up and down along the skateboarding track on Earth. The skater’s motion follows a predictable path: 1. The skater starts from a stationary position. 2. The skater’s direction of motion changes. 3. The downward motion of the skater momentarily becomes horizontal at the bottom of the skating ramp, and then changes direction in an upward direction. 4. The skater nears the top of the skating ramp on the other side, momentarily stops, then changes direction and starts moving again. 5. The downward motion of the skater momentarily becomes horizontal at the bottom of the skating ramp, and then changes direction in an upward direction. 6. The skater reaches the top of the skating ramp where he originally started, momentarily stops, then it resumes a new cycle of movement. Part 1: Exploring Potential Energy 1. Wait until the skateboarder reaches the top part of the ramp and select the Pause button . -
8. Newton's Law Gravitation.Nb
2 8. Newton's Law Gravitation.nb 8. Newton's Law of Gravitation Introduction and Summary There is one other major law due to Newton that will be used in this course and this is his famous Law of Universal Gravitation. It deals with the force between any two massive objects. We will use the Law of Universal Gravitation together with Newton's Laws of Motion to discuss a variety of problems involving the motion of large objects like the Earth moving in orbit about the Sun as well as small objects like the famous apple falling from a tree. Also it will be shown that Newton's 3rd Law of Motion follows as a consequence of Newton's Law of Universal Gravitation. Some additional topics that related to Newton's 1st and 2nd Laws of Motion also will be discussed. In particular, the concept of the Non-Inertia Reference Frame will be introduced and why it is useful. Also it will be shown how Newton's 1st Law and 2nd Law are NOT valid in Non- Inertial Reference Frames. It will be shown what is necessary to "fix-up" Newton's 2nd Law so it works in Non-Inertial Reference Frames. In particular, the examples of accelerated cars and elevators are used to illustrate the concept of the Non-Inertial Frame. The Four Fundamental Forces of Nature: There are four basic or fundamental forces known in nature. These forces are gravity, electromagnetism, the weak nuclear force, and the strong nuclear force. The force of gravity is described by Newton's Law of gravitation and the more recent modification called General Relativity due to Einstein. -
Human Spaceflight. Activities for the Primary Student. Aerospace Education Services Project
DOCUMENT RESUME ED 288 714 SE 048 726 AUTHOR Hartsfield, John W.; Hartsfield, Kendra J. TITLE Human Spaceflight. Activities for the Primary Student. Aerospace Education Services Project. INSTITUTION National Aeronautics and Space Administration, Cleveland, Ohio. Lewis Research Center. PUB DATE Oct 85 NOTE 126p. PUB TYPE Guides - Classroom Use - Materials (For Learner) (051) EDRS PRICE MF01/PC06 Plus Postage. DESCRIPTORS *Aerospace Education; Aerospace Technology; Educational Games; Elementary Education; *Elementary School Science; 'Science Activities; Science and Society; Science Education; *Science History; *Science Instruction; *Space Exploration; Space Sciences IDENTIFIERS *Space Travel ABSTRACT Since its beginning, the space program has caught the attention of young people. This space science activity booklet was designed to provide information and learning activities for students in elementary grades. It contains chapters on:(1) primitive beliefs about flight; (2) early fantasies of flight; (3) the United States human spaceflight programs; (4) a history of human spaceflight activity; (5) life support systems for the astronaut; (6) food for human spaceflight; (7) clothing for spaceflight and activity; (8) warte management systems; (9) a human space flight le;g; and (10) addition 1 activities and pictures. Also included is a bibliography of books, other publications and films, and the answers to the three word puzzles appearing in the booklet. (TW) *********************************************************************** * Reproductions supplied by EDRS are the best that can be made * * from the original document. * *********************************************************************** HUMAN SPACEFLIGHT U.S DEPARTMENT OF EDUCATION Office of Educational Research and Improvement EDUCATIONAL RESOURCES INFORMATION Activities CENTER (ERIC) This document has been reproduced as mewed from the person or organization originating it Minor changes have been made to norm. -
US SENATOR ROBERT C. BYRD's ADDRESSES to June30.I994^ ^Jjg
108 STAT. 5106 CONCURRENT RESOLUTIONS—JUNE 30, 1994 SEC. 2. The Speaker of the House and the majority leader of the Senate, acting jointly after consultation with the minority leader of the House and the minority leader of the Senate, shall notify the Members of the House and the Senate, respectively, to reassemble whenever, in their opinion, the public interest shall warrant it. Agreed to June 30, 1994. T ,n ,00. "U.S. SENATOR ROBERT C. BYRD'S ADDRESSES TO [SJune30.i994. Con. Res. 68^] ^jjg UNITED STATES SENATE ON THE HISTORY OF ROMAN CONSTITUTIONALISM"—SENATE PRINT Resolved by the Senate (the House of Representatives concur ring), That there shall be printed as a Senate document "U.S. Senator Robert C. Byrd's Addresses to the United States Senate on the History of Roman Constitutionalism", delivered between May 5, 1993 and October 18, 1993. SEC. 2. The document referred to in the first section shall be- (1) published under the supervision of the Secretary of the Senate; and (2) in such style, form, manner, and binding as directed by the Joint Committee on Printing, after consultation with the Secretary of the Senate. The document shall include illustrations. SEC. 3. In addition to the usual number of copies of the docu ment, there shall be printed the lesser of— (1) 5,000 copies for the use of the Secretary of the Senate; or (2) such number of copies as does not exceed a total produc tion and printing cost of $47,864. Agreed to June 30, 1994. [Hfr'JirL. Con. Res . -
An Assessment of the Technology of Automated Rendezvous and Capture in Space
National Aeronautics and NASA/TP—1998 –208528 Space Administration AT01S George C. Marshall Space Flight Center Marshall Space Flight Center, Alabama 35812 An Assessment of the Technology of Automated Rendezvous and Capture in Space M.E. Polites Marshall Space Flight Center, Marshall Space Flight Center, Alabama July 1998 The NASA STI Program Office…in Profile Since its founding, NASA has been dedicated to • CONFERENCE PUBLICATION. Collected the advancement of aeronautics and space papers from scientific and technical conferences, science. The NASA Scientific and Technical symposia, seminars, or other meetings sponsored Information (STI) Program Office plays a key or cosponsored by NASA. part in helping NASA maintain this important role. • SPECIAL PUBLICATION. Scientific, technical, or historical information from NASA programs, The NASA STI Program Office is operated by projects, and mission, often concerned with Langley Research Center, the lead center for subjects having substantial public interest. NASA’s scientific and technical information. The NASA STI Program Office provides access to the • TECHNICAL TRANSLATION. NASA STI Database, the largest collection of English-language translations of foreign scientific aeronautical and space science STI in the world. The and technical material pertinent to NASA’s Program Office is also NASA’s institutional mission. mechanism for disseminating the results of its research and development activities. These results Specialized services that complement the STI are published by NASA in the NASA STI Report Program Office’s diverse offerings include creating Series, which includes the following report types: custom thesauri, building customized databases, organizing and publishing research results…even • TECHNICAL PUBLICATION. Reports of providing videos. completed research or a major significant phase of research that present the results of NASA For more information about the NASA STI Program programs and include extensive data or Office, see the following: theoretical analysis.