The Impacts of Large Constellations of Satellites
Total Page:16
File Type:pdf, Size:1020Kb
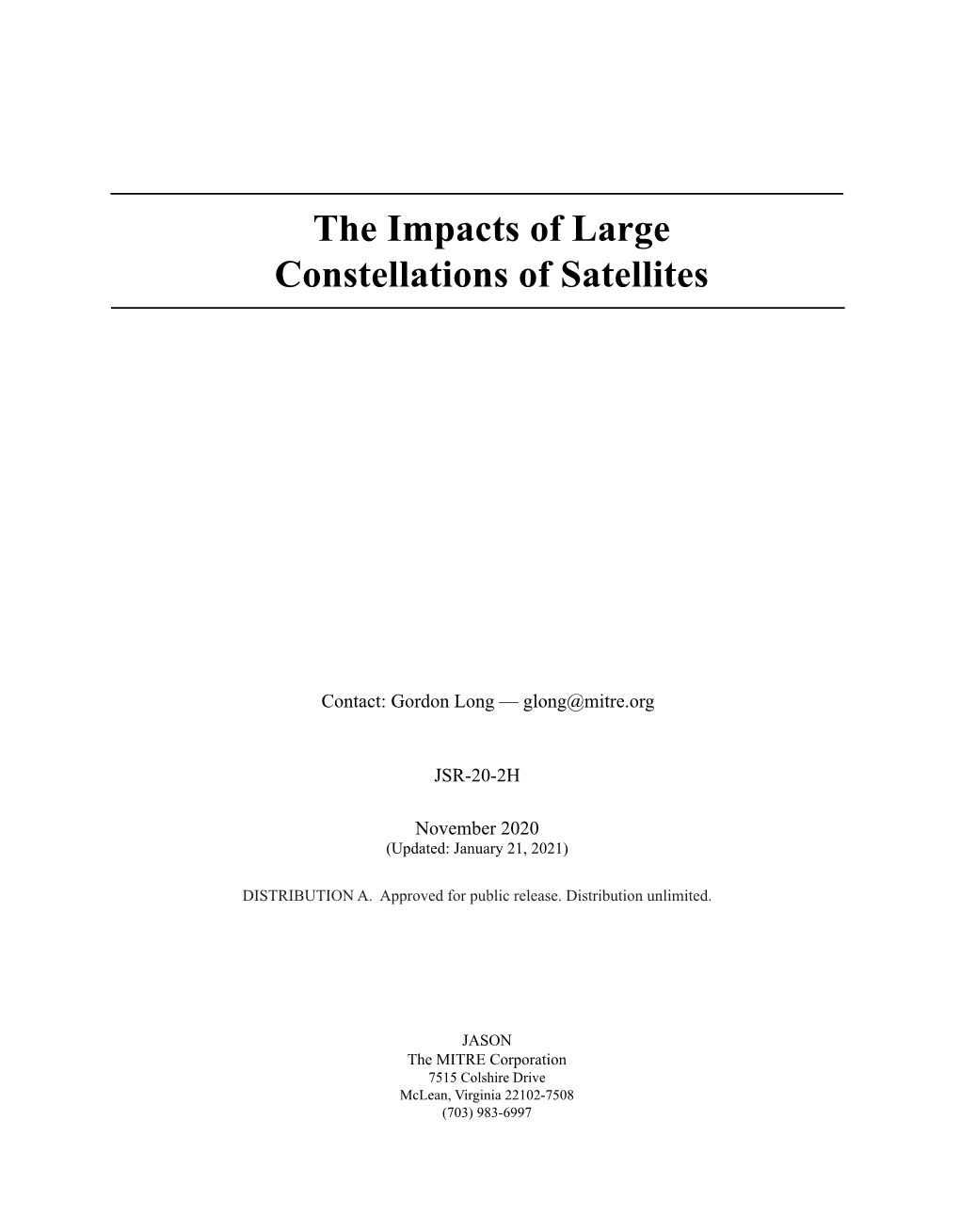
Load more
Recommended publications
-
GT 1020 Datasheet
HARDWARE • DATASHEET GT 1020 Cellular-based telematics for general asset management applications. Complete visibility of transportation and heavy equipment assets, industrial equipment and more. The compact and versatile GT 1020 is designed to fit a wide range of asset tracking 4G LTE cellular applications across various market segments, including transportation, supply communications chain, heavy equipment and maritime. As part of a comprehensive solution that can include cellular connectivity, sensors and a cloud application, the GT 1020 enables remote monitoring of fixed and mobile assets, and delivers actionable data Integrated antennas to ensure complete visibility of operations and enable informed business planning. Feature-rich Quick and easy installation Future proof your solution with global communications over the 4G LTE cellular network with 3G/2G fallback. The GT 1020 features optional Bluetooth support for wireless sensors plus an intelligent reporting system that optimizes power and Compact and rugged airtime usage by adjusting the frequency of location updates based on vehicle motion. An optional CAN Bus interface enables advanced vehicle reporting, analytics and diagnostics, while a backup battery enables reporting for up to 11 Optional CAN Bus support months (depending on reporting frequency) without external power. The device includes a digital input that can be used to report on status changes, such as tire pressure alarms or whether the vehicle is on or off. Feature-rich and versatile Quick and discrete installation Integrated cellular and GPS antennas and mounting options with VHB tape or Long-lasting screws make the GT 1020 quick and easy to install and remove. Its small size backup battery supports covert installations on smaller equipment and assets to deter theft and tampering. -
By Tamman Montanaro
4 Reusable First Stage Rockets y1 = 15.338 m m1 = 2.047 x 10 kg 5 y2 = 5.115 m m2 = 1.613 x 10 kg By Tamman Montanaro What is the moment of inertia? What is the force required from the cold gas thrusters if we assume constancy. Figure 1. Robbert Goddard’s design of the first ever rocket to fly in 1926. Source: George Edward Pendray. The moment of inertia of a solid disk: rper The Rocket Formula Now lets stack a bunch of these solid disk on each other: Length = l Divide by dt Figure 2: Flight path for the Falcon 9; After separation, the first stage orientates itself and prepares itself for landing. Source: SpaceX If we do the same for the hollow cylinder, we get a moment of inertia Launch of: Specific impulse for a rocket: How much mass is lost? What is the mass loss? What is the moment of inertia about the center of mass for these two objects? Divide by m Figure 3: Falcon 9 first stage after landing on drone barge. Source: SpaceX nd On December 22 2015, the Falcon 9 Orbcomm-2 What is the constant force required for its journey halfway (assuming first stage lands successfully. This is the first ever orbital- that the force required to flip it 90o is the equal and opposite to class rocket landing. From the video and flight logs, we Flip Maneuver stabilize the flip). can gather specifications about the first stage. ⃑ How much time does it take for the first stage to descend? We assume this is the time it takes � Flight Specifications for the first stage to reorientate itself. -
Satellite Constellations - 2021 Industry Survey and Trends
[SSC21-XII-10] Satellite Constellations - 2021 Industry Survey and Trends Erik Kulu NewSpace Index, Nanosats Database, Kepler Communications [email protected] ABSTRACT Large satellite constellations are becoming reality. Starlink has launched over 1600 spacecraft in 2 years since the launch of the first batch, Planet has launched over 450, OneWeb more than 200, and counting. Every month new constellation projects are announced, some for novel applications. First part of the paper focuses on the industry survey of 251 commercial satellite constellations. Statistical overview of applications, form factors, statuses, manufacturers, founding years is presented including early stage and cancelled projects. Large number of commercial entities have launched at least one demonstrator satellite, but operational constellations have been much slower to follow. One reason could be that funding is commonly raised in stages and the sustainability of most business models remains to be proven. Second half of the paper examines constellations by selected applications and discusses trends in appli- cations, satellite masses, orbits and manufacturers over the past 5 years. Earliest applications challenged by NewSpace were AIS, Earth Observation, Internet of Things (IoT) and Broadband Internet. Recent years have seen diversification into majority of applications that have been planned or performed by governmental or military satellites, and beyond. INTRODUCTION but they are regarded to be fleets not constellations. There were much fewer Earth Observation com- NewSpace Index has tracked commercial satellite panies in 1990s and 2000s when compared to com- constellations since 2016. There are over 251 entries munications and unclear whether any large constel- as of May 2021, which likely makes it the largest lations were planned. -
Observation of Light Curves of Space Objects Hirohisa Kurosaki Japan
Observation of Light Curves of Space Objects Hirohisa Kurosaki Japan Aerospace Exploration Agency Toshifumi Yanagisawa Japan Aerospace Exploration Agency Atsushi Nakajima Japan Aerospace Exploration Agency Abstract Geostationary orbit and low earth orbit have many artificial satellites, and the accidents of these satellites affect every area such as weather forecasts and communications. In the optical observation, the periodical change of the brightness which is caused by the type and the shape of the satellite is seen. In the case of LEO debris, since the movement is fast, it crosses the field of view in several seconds. We used an observation method to get the data of LEO debris. In case of the GEO debris, the rate of motion is slow in comparison with LEO debris. Fixed stars are observed by the same position on the image when the telescope is operated in sidereal tracking mode. Then, space objects are recorded on the image with streaks of light. In this paper, the observation and the detection method of light curve of geostationary orbit debris are discussed. Observing the changes of the operative satellites with some time intervals may help to detect the abnormalities and accidents of them. 1. INTRODUCTION Artificial satellites that have finished their missions and used rockets are called space debris. Most of them are not under any control. The space debris problem must be solved to perform future space development and maintain safety. Many artificial satellites indispensable for human activities circle the earth. If one of these satellites malfunctions, many human activities are impacted. An artificial satellite is monitored to control its trajectory while it is in operation. -
Ring Road: User Applications on a High Latency Network
User Applications on a High-Latency Network Scott Burleigh 24 January 2014 This research was carried out at the Jet Propulsion Laboratory, California Institute of Technology, under a contract with the National Aeronautics and Space Administration. (c) 2014 California Institute of Technology. Government sponsorship acknowledged. Outline • An infrastructure proposal: a constellation of nanosatellites using delay-tolerant networking to provide low-cost access • An illustration • Some details: capacity, costs • Application latency in this network • Some applications that would work despite the latency • A perspective on using a network • Caveats and outlook 24 January 2014 2 Satellites for Universal Network Access • Earth-orbiting satellites can relay radio communications among sites on Earth. • Can be visible from all points on Earth’s surface, removing geographic and political obstacles. • Not a new idea: – Geostationary (GEO): Exede (ViaSat), HughesNet (EchoStar), WildBlue, StarBand, Intelsat, Inmarsat, Thuraya – Low-Earth Orbiting (LEO): Globalstar, Iridium, Orbcomm, Teledesic 24 January 2014 3 So, Problem Solved? • Maintaining Internet connections with satellites isn’t easy. • GEO satellites do this by ensuring continuous radio contact with ground stations and customer equipment. But: – They are costly, on the order of $300 million (manufacture & launch). – Each one provides communication to a limited part of Earth’s surface. – Each one is a single point of failure. – While data rates are high, round-trip latencies are also high. • LEO constellations do this by constantly switching connections among moving satellites. – Broad coverage areas, low latencies. – But data rates are lower than for GEO, more satellites are needed, and they’re still expensive: $150-$200 million (manufacture and launch). -
INTRODUCTION to U.S. Export Controls for the Commercial Space Industry
INTRODUCTION TO U.S. Export Controls for the Commercial Space Industry 2nd Edition – November 2017 Department of Federal Aviation Commerce Administration This publication was prepared by the U.S. Department of Commerce’s Office of Space Commerce and the Federal Aviation Administration’s Office of Commercial Space Transportation. For additional information, questions, or comments, please visit our websites: www.space.commerce.gov ast.faa.gov IMAGE CREDITS Cover: Spaceflight Industries, Inc. Page 2: Blue Origin Page 12: NASA/Orbital ATK Page 32: Mobilus In Mobili Page 50: Planet Page 58: SSL Page 62: Spire Table of Contents PURPOSE ......................................................................1 SECTION 1: BACKGROUND ...................................................2 1.1 Export Control 101 ........................................................4 1.1.1 Definitions: Exports, Deemed Exports, and Re-exports .................4 1.1.2 The Reasons for Controls ................................................4 1.1.3 Regulations and Responsible Departments .............................5 1.1.4 Multilateral Commitments ..............................................7 1.2 What’s Changed Under Export Control Reform .........................8 1.2.1 Satellite Export Control Reform .........................................8 1.2.2 Major Process Changes under Export Control Reform ..................9 SECTION 2: UNDERSTANDING THE CONTROL LISTS AND HOW THEY WORK ......................................................12 2.1 Overview .................................................................14 -
Spectrum Management: a State of the Profession White Paper
Astro2020 APC White Paper Spectrum Management: A State of the Profession White Paper Type of Activity: ☐ Ground Based Project ☐ Space Based Project ☐ Infrastructure Activity ☐ Technological Development Activity ☒ State of the Profession Consideration ☐ Other Principal Author: Name: Liese van Zee Institution: Indiana University Email: [email protected] Phone: 812 855 0274 Co-authors: (names and institutions) David DeBoer (University of California, Radio Astronomy Lab), Darrel Emerson (Steward Observatory, University of Arizona), Tomas E. Gergely (retired), Namir Kassim (Naval Research Laboratory), Amy J. Lovell (Agnes Scott College), James M. Moran (Center for Astrophysics | Harvard & Smithsonian), Timothy J. Pearson (California Institute of Technology), Scott Ransom (National Radio Astronomy Observatory), and Gregory B. Taylor (University of New Mexico) Abstract (optional): This Astro2020 APC white paper addresses state of the profession considerations regarding spectrum management for the protection of radio astronomy observations. Given the increasing commercial demand for radio spectrum, and the high monetary value associated with such use, innovative approaches to spectrum management will be necessary to ensure the scientific capabilities of current and future radio telescopes. Key aspects include development of methods, in both hardware and software, to improve mitigation and excision of radio frequency interference (RFI). In addition, innovative approaches to radio regulations and coordination between observatories and commercial -
Highlights in Space 2010
International Astronautical Federation Committee on Space Research International Institute of Space Law 94 bis, Avenue de Suffren c/o CNES 94 bis, Avenue de Suffren UNITED NATIONS 75015 Paris, France 2 place Maurice Quentin 75015 Paris, France Tel: +33 1 45 67 42 60 Fax: +33 1 42 73 21 20 Tel. + 33 1 44 76 75 10 E-mail: : [email protected] E-mail: [email protected] Fax. + 33 1 44 76 74 37 URL: www.iislweb.com OFFICE FOR OUTER SPACE AFFAIRS URL: www.iafastro.com E-mail: [email protected] URL : http://cosparhq.cnes.fr Highlights in Space 2010 Prepared in cooperation with the International Astronautical Federation, the Committee on Space Research and the International Institute of Space Law The United Nations Office for Outer Space Affairs is responsible for promoting international cooperation in the peaceful uses of outer space and assisting developing countries in using space science and technology. United Nations Office for Outer Space Affairs P. O. Box 500, 1400 Vienna, Austria Tel: (+43-1) 26060-4950 Fax: (+43-1) 26060-5830 E-mail: [email protected] URL: www.unoosa.org United Nations publication Printed in Austria USD 15 Sales No. E.11.I.3 ISBN 978-92-1-101236-1 ST/SPACE/57 *1180239* V.11-80239—January 2011—775 UNITED NATIONS OFFICE FOR OUTER SPACE AFFAIRS UNITED NATIONS OFFICE AT VIENNA Highlights in Space 2010 Prepared in cooperation with the International Astronautical Federation, the Committee on Space Research and the International Institute of Space Law Progress in space science, technology and applications, international cooperation and space law UNITED NATIONS New York, 2011 UniTEd NationS PUblication Sales no. -
Ice& Stone 2020
Ice & Stone 2020 WEEK 51: DECEMBER 13-19 Presented by The Earthrise Institute # 51 Authored by Alan Hale COMET OF THE WEEK: The Great Comet of 1680 Perihelion: 1680 December 18.49, q = 0.006 AU The Great Comet of 1680 over Rotterdam in The Netherlands, during late December 1680 as painted by the Dutch artist Lieve Verschuier. This particular comet was undoubtedly one of the brightest comets of the 17th Century, but it is also one of the most important comets in history from a scientific perspective, and perhaps even from the perspective of overall human history. While there were certainly plenty of superstitions attached to the comet’s appearance, the scientific investigations made of it were among the beginnings of the era in European history we now call The Enlightenment, and indeed, in a sense the Great Comet of 1680 can perhaps be considered as one of the sparks of that era. The significance began with the comet’s discovery, which was made on the morning of November 14, 1680, by a German astronomer residing in Coburg, Gottfried Kirch – the first comet ever to be discovered by means of a telescope. It was already around 4th magnitude at that time, and located near the star Regulus in the constellation Leo; from that point it traveled eastward and brightened rapidly, being closest to Earth (0.42 AU) on November 30. By that time it was a conspicuous naked-eye object with a tail 20 to 30 degrees long, and it remained visible for another week before disappearing into morning twilight. -
IAC-18-B2.1.7 Page 1 of 16 a Technical Comparison of Three
A Technical Comparison of Three Low Earth Orbit Satellite Constellation Systems to Provide Global Broadband Inigo del Portilloa,*, Bruce G. Cameronb, Edward F. Crawleyc a Department of Aeronautics and Astronautics, Massachusetts Institute of Technology, 77 Massachusetts Avenue, Cambridge 02139, USA, [email protected] b Department of Aeronautics and Astronautics, Massachusetts Institute of Technology, 77 Massachusetts Avenue, Cambridge 02139, USA, [email protected] c Department of Aeronautics and Astronautics, Massachusetts Institute of Technology, 77 Massachusetts Avenue, Cambridge 02139, USA, [email protected] * Corresponding Author Abstract The idea of providing Internet access from space has made a strong comeback in recent years. After a relatively quiet period following the setbacks suffered by the projects proposed in the 90’s, a new wave of proposals for large constellations of low Earth orbit (LEO) satellites to provide global broadband access emerged between 2014 and 2016. Compared to their predecessors, the main differences of these systems are: increased performance that results from the use of digital communication payloads, advanced modulation schemes, multi-beam antennas, and more sophisticated frequency reuse schemes, as well as the cost reductions from advanced manufacturing processes (such as assembly line, highly automated, and continuous testing) and reduced launch costs. This paper compares three such large LEO satellite constellations, namely SpaceX’s 4,425 satellites Ku-Ka-band system, OneWeb’s 720 satellites Ku-Ka-band system, and Telesat’s 117 satellites Ka-band system. First, we present the system architecture of each of the constellations (as described in their respective FCC filings as of September 2018), highlighting the similarities and differences amongst the three systems. -
H. R. 4489 Union Calendar No
1 Union Calendar No. 354 103D CONGRESS 2D SESSION H. R. 4489 [Report No. 103±654] A BILL To authorize appropriations to the National Aero- nautics and Space Administration for human space flight, science, aeronautics, and technology, mission support, and Inspector General, and for other purposes. AUGUST 3, 1994 Reported with an amendment, committed to the Commit- tee of the Whole House on the State of the Union, and ordered to be printed IB Union Calendar No. 354 103D CONGRESS 2D SESSION H. R. 4489 [Report No. 103±654] To authorize appropriations to the National Aeronautics and Space Adminis- tration for human space flight, science, aeronautics, and technology, mission support, and Inspector General, and for other purposes. IN THE HOUSE OF REPRESENTATIVES MAY 25, 1994 Mr. BROWN of California introduced the following bill; which was referred to the Committee on Science, Space, and Technology AUGUST 3, 1994 Reported with an amendment, committed to the Committee of the Whole House on the State of the Union, and ordered to be printed [Strike out all after the enacting clause and insert the part printed in italic] [For text of introduced bill, see copy of bill as introduced on May 25, 1994] A BILL To authorize appropriations to the National Aeronautics and Space Administration for human space flight, science, aeronautics, and technology, mission support, and In- spector General, and for other purposes. 1 Be it enacted by the Senate and House of Representa- 2 tives of the United States of America in Congress assembled, 1 2 1 SECTION 1. SHORT TITLE. 2 This Act may be cited as the ``National Aeronautics 3 and Space Administration Authorization Act, Fiscal Years 4 1995 and 1996''. -
16Th HEAD Meeting Session Table of Contents
16th HEAD Meeting Sun Valley, Idaho – August, 2017 Meeting Abstracts Session Table of Contents 99 – Public Talk - Revealing the Hidden, High Energy Sun, 204 – Mid-Career Prize Talk - X-ray Winds from Black Rachel Osten Holes, Jon Miller 100 – Solar/Stellar Compact I 205 – ISM & Galaxies 101 – AGN in Dwarf Galaxies 206 – First Results from NICER: X-ray Astrophysics from 102 – High-Energy and Multiwavelength Polarimetry: the International Space Station Current Status and New Frontiers 300 – Black Holes Across the Mass Spectrum 103 – Missions & Instruments Poster Session 301 – The Future of Spectral-Timing of Compact Objects 104 – First Results from NICER: X-ray Astrophysics from 302 – Synergies with the Millihertz Gravitational Wave the International Space Station Poster Session Universe 105 – Galaxy Clusters and Cosmology Poster Session 303 – Dissertation Prize Talk - Stellar Death by Black 106 – AGN Poster Session Hole: How Tidal Disruption Events Unveil the High 107 – ISM & Galaxies Poster Session Energy Universe, Eric Coughlin 108 – Stellar Compact Poster Session 304 – Missions & Instruments 109 – Black Holes, Neutron Stars and ULX Sources Poster 305 – SNR/GRB/Gravitational Waves Session 306 – Cosmic Ray Feedback: From Supernova Remnants 110 – Supernovae and Particle Acceleration Poster Session to Galaxy Clusters 111 – Electromagnetic & Gravitational Transients Poster 307 – Diagnosing Astrophysics of Collisional Plasmas - A Session Joint HEAD/LAD Session 112 – Physics of Hot Plasmas Poster Session 400 – Solar/Stellar Compact II 113