Flexible NAD Binding in Deoxyhypusine Synthase Reflects
Total Page:16
File Type:pdf, Size:1020Kb
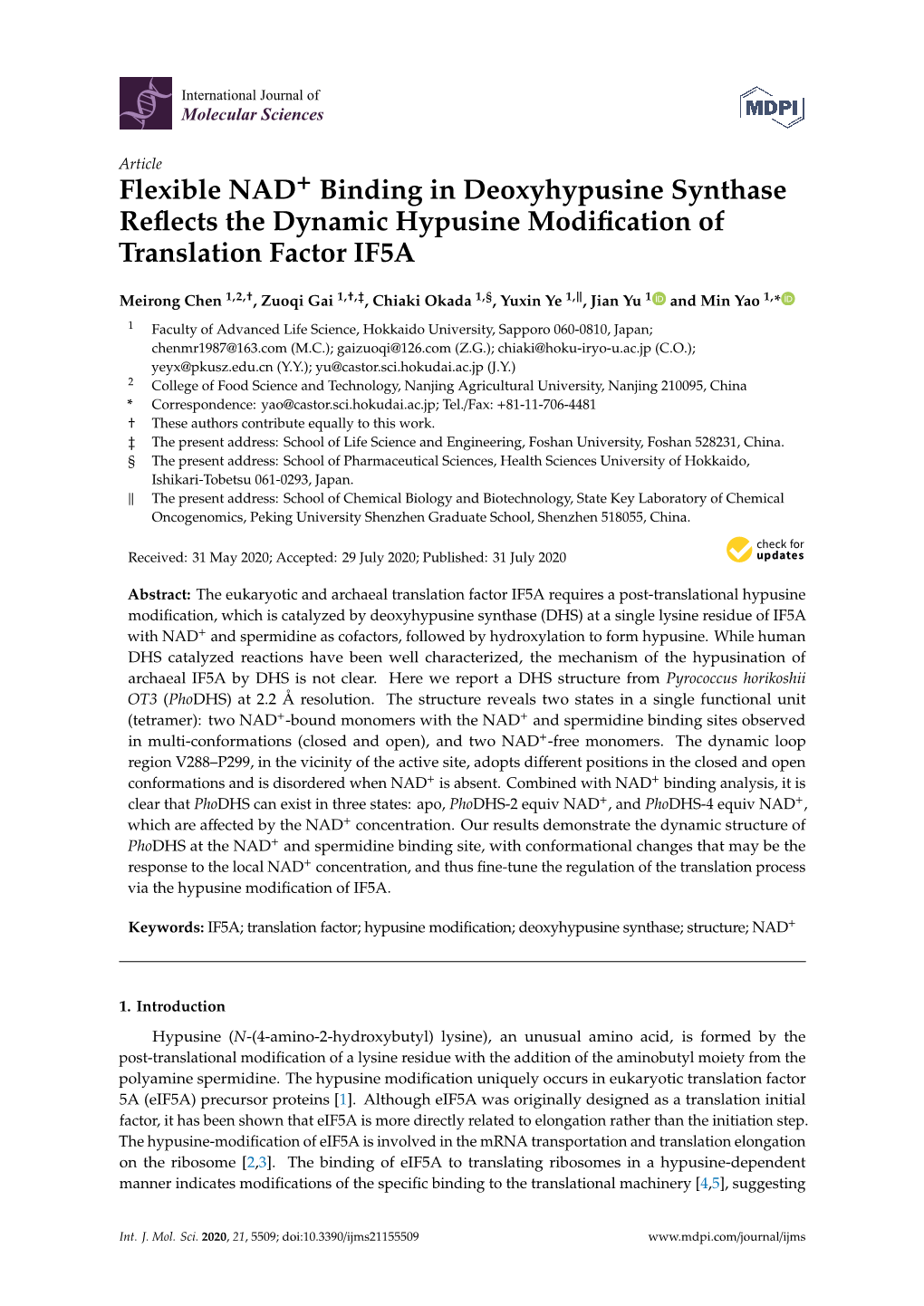
Load more
Recommended publications
-
Hydroxylation of the Eukaryotic Ribosomal Decoding Center Affects Translational Accuracy
Hydroxylation of the eukaryotic ribosomal decoding center affects translational accuracy Christoph Loenarza,1, Rok Sekirnika,2, Armin Thalhammera,2, Wei Gea, Ekaterina Spivakovskya, Mukram M. Mackeena,b,3, Michael A. McDonougha, Matthew E. Cockmanc, Benedikt M. Kesslerb, Peter J. Ratcliffec, Alexander Wolfa,4, and Christopher J. Schofielda,1 aChemistry Research Laboratory and Oxford Centre for Integrative Systems Biology, University of Oxford, Oxford OX1 3TA, United Kingdom; bTarget Discovery Institute, University of Oxford, Oxford OX3 7FZ, United Kingdom; and cCentre for Cellular and Molecular Physiology, University of Oxford, Oxford OX3 7BN, United Kingdom Edited by William G. Kaelin, Jr., Harvard Medical School, Boston, MA, and approved January 24, 2014 (received for review July 31, 2013) The mechanisms by which gene expression is regulated by oxygen Enzyme-catalyzed hydroxylation of intracellularly localized are of considerable interest from basic science and therapeutic proteins was once thought to be rare, but accumulating recent perspectives. Using mass spectrometric analyses of Saccharomyces evidence suggests it is widespread (11). Motivated by these cerevisiae ribosomes, we found that the amino acid residue in findings, we investigated whether the translation of mRNA to closest proximity to the decoding center, Pro-64 of the 40S subunit protein is affected by oxygen-dependent modifications. A rapidly ribosomal protein Rps23p (RPS23 Pro-62 in humans) undergoes growing eukaryotic cell devotes most of its resources to the tran- posttranslational hydroxylation. We identify RPS23 hydroxylases scription, splicing, and transport of ribosomal proteins and rRNA as a highly conserved eukaryotic subfamily of Fe(II) and 2-oxoglu- (12). We therefore reasoned that ribosomal modification is a tarate dependent oxygenases; their catalytic domain is closely re- candidate mechanism for the regulation of protein expression. -
Enzymatic Encoding Methods for Efficient Synthesis Of
(19) TZZ__T (11) EP 1 957 644 B1 (12) EUROPEAN PATENT SPECIFICATION (45) Date of publication and mention (51) Int Cl.: of the grant of the patent: C12N 15/10 (2006.01) C12Q 1/68 (2006.01) 01.12.2010 Bulletin 2010/48 C40B 40/06 (2006.01) C40B 50/06 (2006.01) (21) Application number: 06818144.5 (86) International application number: PCT/DK2006/000685 (22) Date of filing: 01.12.2006 (87) International publication number: WO 2007/062664 (07.06.2007 Gazette 2007/23) (54) ENZYMATIC ENCODING METHODS FOR EFFICIENT SYNTHESIS OF LARGE LIBRARIES ENZYMVERMITTELNDE KODIERUNGSMETHODEN FÜR EINE EFFIZIENTE SYNTHESE VON GROSSEN BIBLIOTHEKEN PROCEDES DE CODAGE ENZYMATIQUE DESTINES A LA SYNTHESE EFFICACE DE BIBLIOTHEQUES IMPORTANTES (84) Designated Contracting States: • GOLDBECH, Anne AT BE BG CH CY CZ DE DK EE ES FI FR GB GR DK-2200 Copenhagen N (DK) HU IE IS IT LI LT LU LV MC NL PL PT RO SE SI • DE LEON, Daen SK TR DK-2300 Copenhagen S (DK) Designated Extension States: • KALDOR, Ditte Kievsmose AL BA HR MK RS DK-2880 Bagsvaerd (DK) • SLØK, Frank Abilgaard (30) Priority: 01.12.2005 DK 200501704 DK-3450 Allerød (DK) 02.12.2005 US 741490 P • HUSEMOEN, Birgitte Nystrup DK-2500 Valby (DK) (43) Date of publication of application: • DOLBERG, Johannes 20.08.2008 Bulletin 2008/34 DK-1674 Copenhagen V (DK) • JENSEN, Kim Birkebæk (73) Proprietor: Nuevolution A/S DK-2610 Rødovre (DK) 2100 Copenhagen 0 (DK) • PETERSEN, Lene DK-2100 Copenhagen Ø (DK) (72) Inventors: • NØRREGAARD-MADSEN, Mads • FRANCH, Thomas DK-3460 Birkerød (DK) DK-3070 Snekkersten (DK) • GODSKESEN, -
Yeast Genome Gazetteer P35-65
gazetteer Metabolism 35 tRNA modification mitochondrial transport amino-acid metabolism other tRNA-transcription activities vesicular transport (Golgi network, etc.) nitrogen and sulphur metabolism mRNA synthesis peroxisomal transport nucleotide metabolism mRNA processing (splicing) vacuolar transport phosphate metabolism mRNA processing (5’-end, 3’-end processing extracellular transport carbohydrate metabolism and mRNA degradation) cellular import lipid, fatty-acid and sterol metabolism other mRNA-transcription activities other intracellular-transport activities biosynthesis of vitamins, cofactors and RNA transport prosthetic groups other transcription activities Cellular organization and biogenesis 54 ionic homeostasis organization and biogenesis of cell wall and Protein synthesis 48 plasma membrane Energy 40 ribosomal proteins organization and biogenesis of glycolysis translation (initiation,elongation and cytoskeleton gluconeogenesis termination) organization and biogenesis of endoplasmic pentose-phosphate pathway translational control reticulum and Golgi tricarboxylic-acid pathway tRNA synthetases organization and biogenesis of chromosome respiration other protein-synthesis activities structure fermentation mitochondrial organization and biogenesis metabolism of energy reserves (glycogen Protein destination 49 peroxisomal organization and biogenesis and trehalose) protein folding and stabilization endosomal organization and biogenesis other energy-generation activities protein targeting, sorting and translocation vacuolar and lysosomal -
Anti-Inflammatory Role of Curcumin in LPS Treated A549 Cells at Global Proteome Level and on Mycobacterial Infection
Anti-inflammatory Role of Curcumin in LPS Treated A549 cells at Global Proteome level and on Mycobacterial infection. Suchita Singh1,+, Rakesh Arya2,3,+, Rhishikesh R Bargaje1, Mrinal Kumar Das2,4, Subia Akram2, Hossain Md. Faruquee2,5, Rajendra Kumar Behera3, Ranjan Kumar Nanda2,*, Anurag Agrawal1 1Center of Excellence for Translational Research in Asthma and Lung Disease, CSIR- Institute of Genomics and Integrative Biology, New Delhi, 110025, India. 2Translational Health Group, International Centre for Genetic Engineering and Biotechnology, New Delhi, 110067, India. 3School of Life Sciences, Sambalpur University, Jyoti Vihar, Sambalpur, Orissa, 768019, India. 4Department of Respiratory Sciences, #211, Maurice Shock Building, University of Leicester, LE1 9HN 5Department of Biotechnology and Genetic Engineering, Islamic University, Kushtia- 7003, Bangladesh. +Contributed equally for this work. S-1 70 G1 S 60 G2/M 50 40 30 % of cells 20 10 0 CURI LPSI LPSCUR Figure S1: Effect of curcumin and/or LPS treatment on A549 cell viability A549 cells were treated with curcumin (10 µM) and/or LPS or 1 µg/ml for the indicated times and after fixation were stained with propidium iodide and Annexin V-FITC. The DNA contents were determined by flow cytometry to calculate percentage of cells present in each phase of the cell cycle (G1, S and G2/M) using Flowing analysis software. S-2 Figure S2: Total proteins identified in all the three experiments and their distribution betwee curcumin and/or LPS treated conditions. The proteins showing differential expressions (log2 fold change≥2) in these experiments were presented in the venn diagram and certain number of proteins are common in all three experiments. -
Amino Acids by GRAHAM C
1 Amino Acids BY GRAHAM C. BARRETT 1 Introduction The literature of 1996 is covered in this Chapter, which aims to report and appraise newly-published knowledge of the chemistry of amino acids. Biological aspects are given prominence only where the chemical interest is enhanced by explaining the life science context. Literature citations forming the basis for this Chapter have been obtained from Chemical Abstracts (Volume 124, Issue no. 11 to Volume 126, Issue no. 9 inclusive), and from papers consulted in major Journals that have consistently been used by authors of relevant material. The expanding volume of the relevant literature continues to demand ingenuity in somehow getting a litre of wholesome nourishment into the half-litre pot that this Chapter represents, and restrictions have been placed on citations of the patent literature and material of a more routine nature. Authors who repeat- publish and over-fragment their material are responsible to a significant extent for the ever-increasing number of references for this Chapter, and this Reviewer’s conscience rests easily when grouping such papers together without detailed comment on each of them. As usual, the carboxylic acid grouping is understood to be implied by the term ‘amino acid’ for the purposes of this Chapter, though interest in boron and phosphorus oxy-acid analogues and also in sulfonic acid analogues, is continuing to grow. Methods applicable .for the synthesis of a-aminoalkaneboronic acids (Refs. 65, 146, 147), a-aminoalkanesulfonic acids (Refs. 154, 845), and a- aminoalkanephosphonic acids and other phosphorus oxyacids (Refs. 32, 62, 80, 82, 85, 87, 88, 152, 326, 374, 437, 843) are usually derived from extensions of standard methods in the amino acid field, and representative examples of syntheses of amino oxyacid analogues are described, side-by-side with corresponding methods for amino carboxylic acids, in appropriate locations in this Chapter. -
Supplementary Table S4. FGA Co-Expressed Gene List in LUAD
Supplementary Table S4. FGA co-expressed gene list in LUAD tumors Symbol R Locus Description FGG 0.919 4q28 fibrinogen gamma chain FGL1 0.635 8p22 fibrinogen-like 1 SLC7A2 0.536 8p22 solute carrier family 7 (cationic amino acid transporter, y+ system), member 2 DUSP4 0.521 8p12-p11 dual specificity phosphatase 4 HAL 0.51 12q22-q24.1histidine ammonia-lyase PDE4D 0.499 5q12 phosphodiesterase 4D, cAMP-specific FURIN 0.497 15q26.1 furin (paired basic amino acid cleaving enzyme) CPS1 0.49 2q35 carbamoyl-phosphate synthase 1, mitochondrial TESC 0.478 12q24.22 tescalcin INHA 0.465 2q35 inhibin, alpha S100P 0.461 4p16 S100 calcium binding protein P VPS37A 0.447 8p22 vacuolar protein sorting 37 homolog A (S. cerevisiae) SLC16A14 0.447 2q36.3 solute carrier family 16, member 14 PPARGC1A 0.443 4p15.1 peroxisome proliferator-activated receptor gamma, coactivator 1 alpha SIK1 0.435 21q22.3 salt-inducible kinase 1 IRS2 0.434 13q34 insulin receptor substrate 2 RND1 0.433 12q12 Rho family GTPase 1 HGD 0.433 3q13.33 homogentisate 1,2-dioxygenase PTP4A1 0.432 6q12 protein tyrosine phosphatase type IVA, member 1 C8orf4 0.428 8p11.2 chromosome 8 open reading frame 4 DDC 0.427 7p12.2 dopa decarboxylase (aromatic L-amino acid decarboxylase) TACC2 0.427 10q26 transforming, acidic coiled-coil containing protein 2 MUC13 0.422 3q21.2 mucin 13, cell surface associated C5 0.412 9q33-q34 complement component 5 NR4A2 0.412 2q22-q23 nuclear receptor subfamily 4, group A, member 2 EYS 0.411 6q12 eyes shut homolog (Drosophila) GPX2 0.406 14q24.1 glutathione peroxidase -
Amino Acids by G.C.BARRETT
1 Amino Acids By G.C.BARRETT 1 Introduction The chemistry and biochemistry of the amino acids as represented in the 1992 literature, is covered in this Chapter. The usual policy for this Specialist Periodical Report has been continued, with almost exclusive attention in this Chapter, to the literature covering the natural occurrence, chemistry, and analysis methodology for the amino acids. Routine literature covering the natural distribution of well-known amino acids is excluded. The discussion offered is brief for most of the papers cited, so that adequate commentary can be offered for papers describing significant advances in synthetic methodology and mechanistically-interesting chemistry. Patent literature is almost wholly excluded but this is easily reached through Section 34 of Chemical Abstracts. It is worth noting that the relative number of patents carried in Section 34 of Chemical Abstracts is increasing (e.g. Section 34 of Chem-Abs., 1992, Vol. 116, Issue No. 11 contains 45 patent abstracts, 77 abstracts of papers and reviews), reflecting the perception that amino acids and peptides are capable of returning rich commercial rewards due to their important physiological roles and consequent pharmaceutical status. However, there is no slowing of the flow of journal papers and secondary literature, as far as the amino acids are concerned. The coverage in this Chapter is arranged into sections as used in all previous Volumes of this Specialist Periodical Report, and major Journals and Chemical Abstracts (to Volume 118, issue 11) have -
Conditioning Medicine
Conditioning Medicine www.conditionmed.org REVIEW ARTICLE | OPEN ACCESS A new pharmacological preconditioning-based target: from drosophila to kidney transplantation Michel Tauc1*, Nicolas Melis1*, Miled Bourourou2, Sébastien Giraud3, Thierry Hauet3, and Nicolas Blondeau2 One of the biggest challenges in medicine is to dampen the pathophysiological stress induced by an episode of ischemia. Such stress, due to various pathological or clinical situations, follows a restriction in blood and oxygen supply to tissue, causing a shortage of oxygen and nutrients that are required for cellular metabolism. Ischemia can cause irreversible damage to target tissue leading to a poor physiological recovery outcome for the patient. Contrariwise, preconditioning by brief periods of ischemia has been shown in multiple organs to confer tolerance against subsequent normally lethal ischemia. By definition, preconditioning of organs must be applied preemptively. This limits the applicability of preconditioning in clinical situations, which arise unpredictably, such as myocardial infarction and stroke. There are, however, clinical situations that arise as a result of ischemia-reperfusion injury, which can be anticipated, and are therefore adequate candidates for preconditioning. Organ and more particularly kidney transplantation, the optimal treatment for suitable patients with end stage renal disease (ESRD), is a predictable surgery that permits the use of preconditioning protocols to prepare the organ for subsequent ischemic/reperfusion stress. It therefore seems crucial to develop appropriate preconditioning protocols against ischemia that will occur under transplantation conditions, which up to now mainly referred to mechanical ischemic preconditioning that triggers innate responses. It is not known if preconditioning has to be applied to the donor, the recipient, or both. -
Roles of Eukaryotic Initiation Factor 5A2 in Human Cancer Feng-Wei Wang1, Xin-Yuan Guan2, Dan Xie1
Int. J. Biol. Sci. 2013, Vol. 9 1013 Ivyspring International Publisher International Journal of Biological Sciences 2013; 9(10):1013-1020. doi: 10.7150/ijbs.7191 Review Roles of Eukaryotic Initiation Factor 5A2 in Human Cancer Feng-wei Wang1, Xin-yuan Guan2, Dan Xie1 1. Sun Yat-sen University Cancer Center; State Key Laboratory of Oncology in South China. Collaborative Innovation Center of Cancer Medicine. 2. Department of Clinical Oncology, the University of Hong Kong, Hong Kong, China. Corresponding author: Dan Xie, M.D. Ph.D. Sun Yat-Sen University Cancer Center, State key laboratory of oncology in South China, Collaborative Innovation Center of Cancer Medicine, No. 651, Dongfeng Road East, 510060 Guangzhou, China. Tel: 86-20-87343192 Fax: 86-20-87343170 Email: [email protected]. © Ivyspring International Publisher. This is an open-access article distributed under the terms of the Creative Commons License (http://creativecommons.org/ licenses/by-nc-nd/3.0/). Reproduction is permitted for personal, noncommercial use, provided that the article is in whole, unmodified, and properly cited. Received: 2013.07.18; Accepted: 2013.09.26; Published: 2013.10.12 Abstract Eukaryotic initiation factor 5A (eIF5A), the only known cellular protein containing the amino acid hypusine, is an essential component of translation elongation. eIF5A2, one of the two isoforms in the eIF5A family, is reported to be a novel oncogenic protein in many types of human cancer. Both in vitro and in vivo studies showed that eIF5A2 could initiate tumor formation, enhance cancer cell growth, and increase cancer cell motility and metastasis by inducing epithelial-mesenchymal transition. -
The Development and Improvement of Instructions
INITIAL CHARACTERIZATION OF HUMAN SPERMINE OXIDASE A Thesis by PAUL RAMON JUAREZ Submitted to the Office of Graduate Studies of Texas A&M University in partial fulfillment of the requirements for the degree of MASTER OF SCIENCE December 2008 Major Subject: Biochemistry INITIAL CHARACTERIZATION OF HUMAN SPERMINE OXIDASE A Thesis by PAUL RAMON JUAREZ Submitted to the Office of Graduate Studies of Texas A&M University in partial fulfillment of the requirements for the degree of MASTER OF SCIENCE Approved by: Chair of Committee, Paul F. Fitzpatrick Committee Members, Dorothy Shippen J. Martin Scholtz Head of Department, Gregory D. Reinhart December 2008 Major Subject: Biochemistry iii ABSTRACT Initial Characterization of Human Spermine Oxidase. (December 2008) Paul Ramon Juarez, B.S.; B.A., Texas A&M University-Corpus Christi Chair of Advisory Committee: Dr. Paul F. Fitzpatrick The flavoprotein spermine oxidase catalyzes the oxidation of spermine and oxygen to spermidine, 3-aminopropanol, and hydrogen peroxide. To allow mechanistic studies of the enzyme, methods have been developed to obtain large amounts of purified recombinant protein. The enzyme requires co-expression with chaperone proteins GroEL and GroES to remain soluble and active. Purification requires the use of a Ni- NTA and size exclusion column. Human spermine oxidase is a monomer with an extinction coefficient of 14000 M-1cm-1. The kinetic mechanism is ping pong. Therefore, oxygen is bound to the enzyme before spermidine is released. N1-Acetyl spermine is a slow substrate with kcat and kcat/Km values 2 and 3 orders of magnitude smaller than the values for spermine. Spermidine is a competitive inhibitor, and 1,8- diaminooctane (DAO) is an uncompetitive inhibitor. -
A Novel Mouse Model for Inhibition of DOHH-Mediated Hypusine Modification Reveals a Crucial Function in Embryonic Development, P
© 2014. Published by The Company of Biologists Ltd | Disease Models & Mechanisms (2014) 7, 963-976 doi:10.1242/dmm.014449 RESEARCH ARTICLE A novel mouse model for inhibition of DOHH-mediated hypusine modification reveals a crucial function in embryonic development, proliferation and oncogenic transformation Henning Sievert1, Nora Pällmann1,2,*, Katharine K. Miller3,*, Irm Hermans-Borgmeyer3, Simone Venz4, Ataman Sendoel5,6, Michael Preukschas1, Michaela Schweizer3, Steffen Boettcher6, P. Christoph Janiesch3, Thomas Streichert7, Reinhard Walther4, Michael O. Hengartner5, Markus G. Manz6, Tim H. Brümmendorf8, Carsten Bokemeyer1, Melanie Braig1, Joachim Hauber2, Kent E. Duncan3 and Stefan Balabanov1,6,‡ ABSTRACT factor 5A (eIF5A), represents an essential mechanism in the control The central importance of translational control by post-translational of proliferation of eukaryotic cells (Cooper et al., 1982). This modification has spurred major interest in regulatory pathways that modification leads to the activation of eIF5A and is mediated by control translation. One such pathway uniquely adds hypusine to deoxyhypusine synthase (DHS), which catalyses the transfer of a 4- eukaryotic initiation factor 5A (eIF5A), and thereby affects protein aminobutyl moiety of spermidine to the ε-amino group of Lys50 to synthesis and, subsequently, cellular proliferation through an form an intermediate residue, deoxyhypusine (Dhp50) (Park et al., unknown mechanism. Using a novel conditional knockout mouse 1981). Subsequently, deoxyhypusine hydroxylase (DOHH) -
Function of Elongation Factor P in Translation
Function of Elongation Factor P in Translation Dissertation for the award of the degree ”Doctor rerum naturalium“ of the Georg-August-Universität Göttingen within the doctoral program Biomolecules: Structure–Function–Dynamics of the Georg-August University School of Science (GAUSS) submitted by Lili Klara Dörfel from Berlin Göttingen, 2015 Members of the Examination board / Thesis Committee Prof. Dr. Marina Rodnina, Department of Physical Biochemistry, Max Planck Institute for Biophysical Chemistry, Göttingen (1st Reviewer) Prof. Dr. Heinz Neumann, Research Group of Applied Synthetic Biology, Institute for Microbiology and Genetics, Georg August University, Göttingen (2nd Reviewer) Prof. Dr. Holger Stark, Research Group of 3D Electron Cryo-Microscopy, Max Planck Institute for Biophysical Chemistry, Göttingen Further members of the Examination board Prof. Dr. Ralf Ficner, Department of Molecular Structural Biology, Institute for Microbiology and Genetics, Georg August University, Göttingen Dr. Manfred Konrad, Research Group Enzyme Biochemistry, Max Planck Institute for Biophysical Chemistry, Göttingen Prof. Dr. Markus T. Bohnsack, Department of Molecular Biology, Institute for Molecular Biology, University Medical Center, Göttingen Date of the oral examination: 16.11.2015 I Affidavit The thesis has been written independently and with no other sources and aids than quoted. Sections 2.1.2, 2.2.1.1 and parts of section 2.2.1.4 are published in (Doerfel et al, 2013); the translation gel of EspfU is published in (Doerfel & Rodnina, 2013) and section 2.2.3 is published in (Doerfel et al, 2015) (see list of publications). Lili Klara Dörfel November 2015 II List of publications EF-P is Essential for Rapid Synthesis of Proteins Containing Consecutive Proline Residues Doerfel LK†, Wohlgemuth I†, Kothe C, Peske F, Urlaub H, Rodnina MV*.