Transgenic Archaerhodopsin-3 Expression in Hypocretin/Orexin
Total Page:16
File Type:pdf, Size:1020Kb
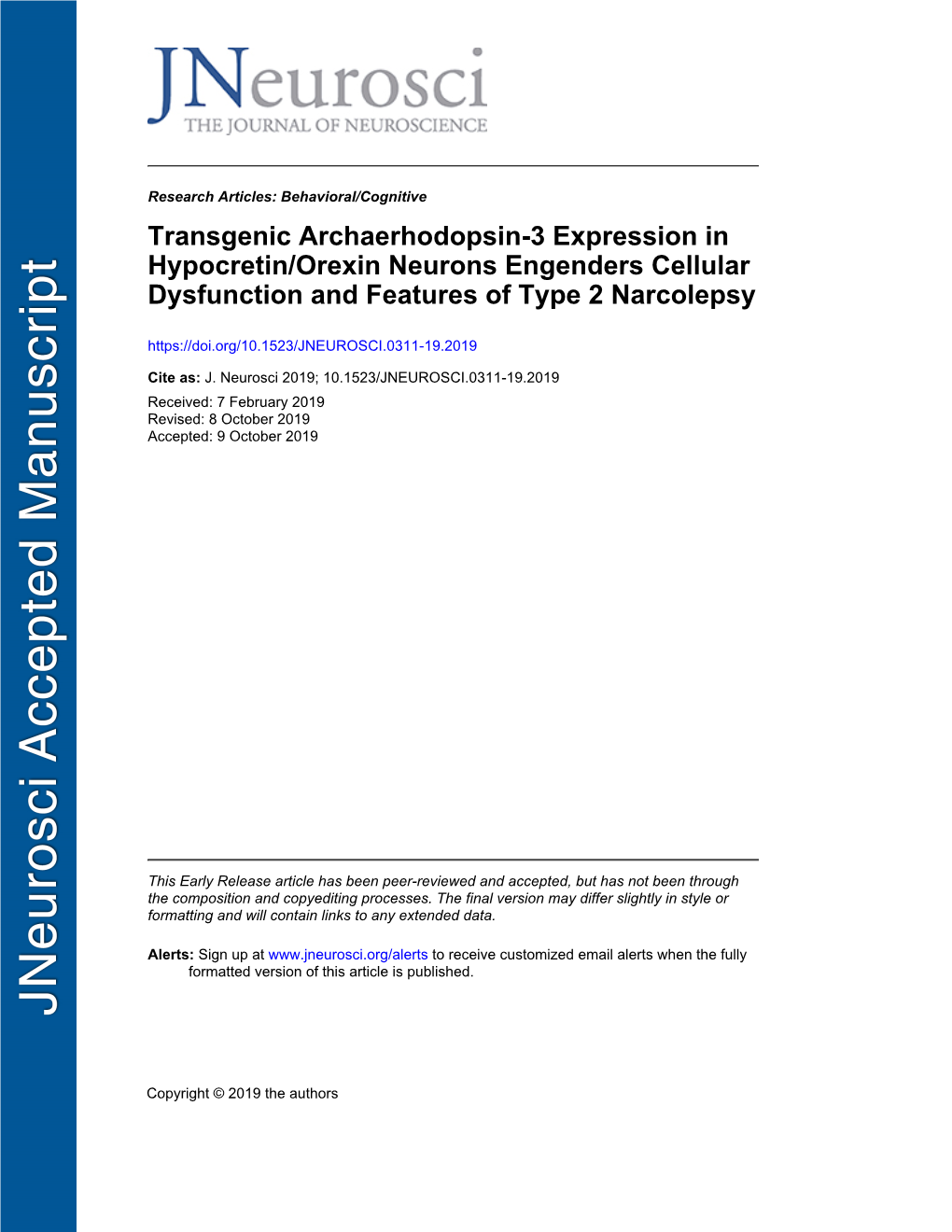
Load more
Recommended publications
-
A Voltage-Dependent Fluorescent Indicator for Optogenetic Applications, Archaerhodopsin-3: Structure and Optical Properties
F1000Research 2017, 6:33 Last updated: 30 JUL 2021 RESEARCH NOTE A voltage-dependent fluorescent indicator for optogenetic applications, archaerhodopsin-3: Structure and optical properties from in silico modeling [version 3; peer review: 3 approved] Dmitrii M. Nikolaev1, Anton Emelyanov1, Vitaly M. Boitsov1, Maxim S Panov2, Mikhail N. Ryazantsev 2,3 1Saint-Petersburg National Research Academic University of the Russian Academy of Science, St. Petersburg, Russian Federation 2Saint-Petersburg State University, St. Petersburg, Russian Federation 3Saint-Petersburg Scientific Center of the Russian Academy of Sciences, St. Petersburg, Russian Federation v3 First published: 11 Jan 2017, 6:33 Open Peer Review https://doi.org/10.12688/f1000research.10541.1 Second version: 17 Jan 2017, 6:33 https://doi.org/10.12688/f1000research.10541.2 Reviewer Status Latest published: 15 Nov 2017, 6:33 https://doi.org/10.12688/f1000research.10541.3 Invited Reviewers 1 2 3 Abstract It was demonstrated in recent studies that some rhodopsins can be version 3 used in optogenetics as fluorescent indicators of membrane voltage. (revision) report report One of the promising candidates for these applications is 15 Nov 2017 archaerhodopsin-3. While it has already shown encouraging results, there is still a large room for improvement. One of possible directions version 2 is increasing the intensity of the protein's fluorescent signal. Rational (revision) report report report design of mutants with an improved signal is an important task, which 17 Jan 2017 requires both experimental and theoretical studies. Herein, we used a homology-based computational approach to predict the three- version 1 dimensional structure of archaerhodopsin-3, and a Quantum 11 Jan 2017 Mechanics/Molecular Mechanics (QM/MM) hybrid approach with high- level multireference ab initio methodology (SORCI+Q/AMBER) to 1. -
Han-Optogenetic-Review-ACS-2012.Pdf
Review pubs.acs.org/chemneuro In Vivo Application of Optogenetics for Neural Circuit Analysis Xue Han Biomedical Engineering Department, Boston University, Boston, Massachusetts, United States ABSTRACT: Optogenetics combines optical and genetic methods to rapidly and reversibly control neural activities or other cellular functions. Using genetic methods, specific cells or anatomical pathways can be sensitized to light through exogenous expression of microbial light activated opsin proteins. Using optical methods, opsin expressing cells can be rapidly and reversibly controlled by pulses of light of specific wavelength. With the high spatial temporal precision, optogenetic tools have enabled new ways to probe the causal role of specific cells in neural computation and behavior. Here, we overview the current state of the technology, and provide a brief introduction to the practical considerations in applying optogenetics in vivo to analyze neural circuit functions. KEYWORDS: Channelrhodopsin, archaerhodopsin, halorhodopsin, cell type specificity ptogenetics is a new field being rapidly established upon algae), and others that mediate a variety of cellular functions O the first demonstration of precise activation of neurons (for reviews on opsin structure and function, see refs 9 and 10). expressing a light-activated microbial opsin, channelrhodopsin- Most sensory rhodopsins function through recruiting intra- 2, with pulses of blue light in 2005.1 Microbial (type I) opsins cellular signaling molecules without direct ion transport are classes of monolithic light activated proteins, encoded by function. However, channelrhodopsins can mediate phototaxis small genes of under a kilobase long. Three major classes of as a light-gated cation channel at high light intensity and as a microbial opsins have been adapted to optogenetically control calcium channel at low light intensity.11 cellular functions, channelrhodopsins, halorhodopsins, and Microbial opsins share sequence homology and are archaerhodopsins (Figure 1). -
High-Performance Genetically Targetable Optical Neural Silencing by Light-Driven Proton Pumps
Vol 463 | 7 January 2010 | doi:10.1038/nature08652 LETTERS High-performance genetically targetable optical neural silencing by light-driven proton pumps Brian Y. Chow1,2*, Xue Han1,2*, Allison S. Dobry1,2, Xiaofeng Qian1,2, Amy S. Chuong1,2, Mingjie Li1,2, Michael A. Henninger1,2, Gabriel M. Belfort2, Yingxi Lin2, Patrick E. Monahan1,2 & Edward S. Boyden1,2 The ability to silence the activity of genetically specified neurons in screen, as did two other proton pumps, the Leptosphaeria maculans a temporally precise fashion would provide the opportunity to opsin (Mac/LR/Ops)4 and cruxrhodopsin-1 (ref. 10) (albeit less than investigate the causal role of specific cell classes in neural compu- that of Arch; Fig. 1a). All light-driven chloride pumps assessed had tations, behaviours and pathologies. Here we show that members lower screen photocurrents than these light-driven proton pumps. of the class of light-driven outward proton pumps can mediate Arch is a yellow–green light-sensitive (Fig. 1b) opsin that seems to powerful, safe, multiple-colour silencing of neural activity. The express well on the neural plasma membrane (Fig. 1c; see Sup- gene archaerhodopsin-3 (Arch)1 from Halorubrum sodomense plementary Notes on Arch expression levels and enhancing Arch enables near-100% silencing of neurons in the awake brain when membrane trafficking). Arch-mediated currents exhibited excellent virally expressed in the mouse cortex and illuminated with yellow kinetics of light-activation and post-light recovery. After illumination, light. Arch mediates currents of several hundred picoamps at low Arch currents rose with a 15–85% onset time of 8.8 6 1.8 ms light powers, and supports neural silencing currents approaching (mean 6 standard error (s.e.) reported throughout, unless otherwise 900 pA at light powers easily achievable in vivo. -
Illuminating Brain Activities with Fluorescent Protein-Based Biosensors
chemosensors Review Illuminating Brain Activities with Fluorescent Protein-Based Biosensors Zhijie Chen 1 ID , Tan M. Truong 2 and Hui-wang Ai 2,3,4,* ID 1 California Institute for Quantitative Biosciences, QB3, University of California, Berkeley, CA 94720, USA; [email protected] 2 Center for Membrane and Cell Physiology, and Biomedical Sciences (BIMS) Graduate Program, University of Virginia, Charlottesville, VA 22908, USA; [email protected] 3 Department of Molecular Physiology and Biological Physics, University of Virginia, Charlottesville, VA 22908, USA 4 Department of Chemistry, University of Virginia, Charlottesville, VA 22908, USA * Correspondence: [email protected] Received: 9 October 2017; Accepted: 22 November 2017; Published: 28 November 2017 Abstract: Fluorescent protein-based biosensors are indispensable molecular tools for life science research. The invention and development of high-fidelity biosensors for a particular molecule or molecular event often catalyze important scientific breakthroughs. Understanding the structural and functional organization of brain activities remain a subject for which optical sensors are in desperate need and of growing interest. Here, we review genetically encoded fluorescent sensors for imaging neuronal activities with a focus on the design principles and optimizations of various sensors. New bioluminescent sensors useful for deep-tissue imaging are also discussed. By highlighting the protein engineering efforts and experimental applications of these sensors, we can consequently analyze factors influencing their performance. Finally, we remark on how future developments can fill technological gaps and lead to new discoveries. Keywords: fluorescent proteins; biosensors; fluorescent probes/indicators; brain imaging; genetically encoded calcium indicators; genetically encoded voltage sensors; genetically encoded glutamate sensors; synaptic activity indicators; pH indicators; bioluminescent sensors 1. -
Lokiarchaeota Archaeon Schizorhodopsin-2 (Laszr2) Is an Inward Proton Pump Displaying a Characteristic Feature of Acid-Induced S
www.nature.com/scientificreports OPEN Lokiarchaeota archaeon schizorhodopsin‑2 (LaSzR2) is an inward proton pump displaying a characteristic feature of acid‑induced spectral blue‑shift Keiichi Kojima1,5, Susumu Yoshizawa2,5, Masumi Hasegawa2, Masaki Nakama1, Marie Kurihara1, Takashi Kikukawa3,4 & Yuki Sudo1,2* The photoreactive protein rhodopsin is widespread in microorganisms and has a variety of photobiological functions. Recently, a novel phylogenetically distinctive group named ‘schizorhodopsin (SzR)’ has been identifed as an inward proton pump. We performed functional and spectroscopic studies on an uncharacterised schizorhodopsin from the phylum Lokiarchaeota archaeon. The protein, LaSzR2, having an all‑trans-retinal chromophore, showed inward proton pump activity with an absorption maximum at 549 nm. The pH titration experiments revealed that the protonated Schif base of the retinal chromophore (Lys188, pKa = 12.3) is stabilised by the deprotonated counterion (presumably Asp184, pKa = 3.7). The fash‑photolysis experiments revealed the presence of two photointermediates, K and M. A proton was released and uptaken from bulk solution upon the formation and decay of the M intermediate. During the M‑decay, the Schif base was reprotonated by the proton from a proton donating residue (presumably Asp172). These properties were compared with other inward (SzRs and xenorhodopsins, XeRs) and outward proton pumps. Notably, LaSzR2 showed acid‑induced spectral ‘blue‑shift’ due to the protonation of the counterion, whereas outward proton pumps showed opposite shifts (red‑shifts). Thus, we can distinguish between inward and outward proton pumps by the direction of the acid‑induced spectral shift. Sunlight provides a fundamental source for organisms, including humans. Many organisms possess a variety of photoreceptor proteins that can capture sunlight. -
Atomistic Design of Microbial Opsin-Based Blue-Shifted Optogenetics Tools
ARTICLE Received 4 Nov 2014 | Accepted 14 Apr 2015 | Published 15 May 2015 DOI: 10.1038/ncomms8177 OPEN Atomistic design of microbial opsin-based blue-shifted optogenetics tools Hideaki E. Kato1,*,w, Motoshi Kamiya2,*, Seiya Sugo2, Jumpei Ito3, Reiya Taniguchi1, Ayaka Orito3, Kunio Hirata4, Ayumu Inutsuka5, Akihiro Yamanaka5, Andre´s D. Maturana3, Ryuichiro Ishitani1, Yuki Sudo6, Shigehiko Hayashi2 & Osamu Nureki1 Microbial opsins with a bound chromophore function as photosensitive ion transporters and have been employed in optogenetics for the optical control of neuronal activity. Molecular engineering has been utilized to create colour variants for the functional augmentation of optogenetics tools, but was limited by the complexity of the protein–chromophore inter- actions. Here we report the development of blue-shifted colour variants by rational design at atomic resolution, achieved through accurate hybrid molecular simulations, electrophysiology and X-ray crystallography. The molecular simulation models and the crystal structure reveal the precisely designed conformational changes of the chromophore induced by combinatory mutations that shrink its p-conjugated system which, together with electrostatic tuning, produce large blue shifts of the absorption spectra by maximally 100 nm, while maintaining photosensitive ion transport activities. The design principle we elaborate is applicable to other microbial opsins, and clarifies the underlying molecular mechanism of the blue-shifted action spectra of microbial opsins recently isolated from natural sources. 1 Department of Biological Sciences, Graduate School of Science, The University of Tokyo, 2-11-16 Yayoi, Bunkyo-ku, Tokyo 113-0032, Japan. 2 Department of Chemistry, Graduate School of Science, Kyoto University, Kyoto 606-8502, Japan. 3 Department of Bioengineering Sciences, Graduate School of Bioagricultural Sciences, Nagoya University, Furo-cho, Chikusa-ku, Nagoya 464-8601, Japan. -
Bright and Fast Multicoloured Voltage Reporters Via Electrochromic FRET
ARTICLE Received 18 Mar 2014 | Accepted 8 Jul 2014 | Published 13 Aug 2014 DOI: 10.1038/ncomms5625 Bright and fast multicoloured voltage reporters via electrochromic FRET Peng Zou1,*, Yongxin Zhao2,*, Adam D. Douglass3, Daniel R. Hochbaum1, Daan Brinks1, Christopher A. Werley1, D. Jed Harrison2, Robert E. Campbell2 & Adam E. Cohen1,4 Genetically encoded fluorescent reporters of membrane potential promise to reveal aspects of neural function not detectable by other means. We present a palette of multicoloured brightly fluorescent genetically encoded voltage indicators with sensitivities from 8–13% DF/F per 100 mV, and half-maximal response times from 4–7 ms. A fluorescent protein is fused to an archaerhodopsin-derived voltage sensor. Voltage-induced shifts in the absorption spectrum of the rhodopsin lead to voltage-dependent nonradiative quenching of the appended fluorescent protein. Through a library screen, we identify linkers and fluorescent protein combinations that report neuronal action potentials in cultured rat hippocampal neurons with a single-trial signal-to-noise ratio from 7 to 9 in a 1 kHz imaging bandwidth at modest illumination intensity. The freedom to choose a voltage indicator from an array of colours facilitates multicolour voltage imaging, as well as combination with other optical reporters and optogenetic actuators. 1 Departments of Chemistry and Chemical Biology and Physics, Harvard Stem Cell Institute, Harvard University, Cambridge, Massachusetts 02138, USA. 2 Department of Chemistry, University of Alberta, Edmonton, Alberta, Canada T6G 2G2. 3 Department of Neurobiology and Anatomy, University of Utah, Salt Lake City, Utah 84132, USA. 4 Howard Hughes Medical Institute, Harvard University, 12 Oxford Street, Cambridge, Massachusetts 02138, USA. -
Crystal Structure of the Natural Anion-Conducting
ARTICLE https://doi.org/10.1038/s41586-018-0511-6 Crystal structure of the natural anion- conducting channelrhodopsin GtACR1 Yoon Seok Kim1,9, Hideaki E. Kato2,3,9*, Keitaro Yamashita4, Shota Ito5, Keiichi Inoue3,5,6, Charu Ramakrishnan1, Lief E. Fenno1, Kathryn E. Evans1, Joseph M. Paggi7,8, Ron O. Dror7,8, Hideki Kandori5,6, Brian K. Kobilka2 & Karl Deisseroth1* The naturally occurring channelrhodopsin variant anion channelrhodopsin-1 (ACR1), discovered in the cryptophyte algae Guillardia theta, exhibits large light-gated anion conductance and high anion selectivity when expressed in heterologous settings, properties that support its use as an optogenetic tool to inhibit neuronal firing with light. However, molecular insight into ACR1 is lacking owing to the absence of structural information underlying light-gated anion conductance. Here we present the crystal structure of G. theta ACR1 at 2.9 Å resolution. The structure reveals unusual architectural features that span the extracellular domain, retinal-binding pocket, Schiff-base region, and anion-conduction pathway. Together with electrophysiological and spectroscopic analyses, these findings reveal the fundamental molecular basis of naturally occurring light-gated anion conductance, and provide a framework for designing the next generation of optogenetic tools. Most organisms depend on light for energy and information. Motile naturally occurring ACRs were isolated from chlorophyte algae21 organisms typically capture light using rhodopsin proteins, largely (GtACR1 and GtACR2). The designed ACRs have been developed classified into two groups: microbial (type I) and animal (type II)1,2, further22–24, and additional natural ACRs have been found by genome both exhibiting seven-transmembrane helices and a retinal-based mining25–27. -
Optogenetic Manipulation of Individual Or Whole Population Caenorhabditis Elegans Worms with an Under Hundred-Dollar Tool
bioRxiv preprint doi: https://doi.org/10.1101/2021.03.19.435933; this version posted March 22, 2021. The copyright holder for this preprint (which was not certified by peer review) is the author/funder, who has granted bioRxiv a license to display the preprint in perpetuity. It is made available under aCC-BY-NC-ND 4.0 International license. 1 Optogenetic manipulation of individual or whole population 2 Caenorhabditis elegans worms with an under hundred-dollar tool: 3 the OptoArm. 4 5 M. Koopman1*, L. Janssen1, E.A.A. Nollen1* 6 1European Research Institute for the Biology of Ageing, University of Groningen, University Medical Centre 7 Groningen, The Netherlands 8 *Corresponding authors: [email protected] or [email protected] 9 10 Abstract 11 Optogenetic tools have revolutionized the study of neuronal circuits in Caenorhabditis elegans. The expression of 12 light-sensitive ion channels or pumps under specific promotors allows researchers to modify the behavior of 13 excitable cells. Several optogenetic systems have been developed to spatially and temporally photoactivate light- 14 sensitive actuators in C. elegans. Nevertheless, their high costs and low flexibility have limited wide access to 15 optogenetics. Here, we developed an inexpensive, easy-to-build, and adjustable optogenetics device for use on 16 different microscopes and worm trackers, called the OptoArm. The OptoArm allows for single- and multiple- 17 worm illumination and is adaptable in terms of light intensity, lighting profiles and light-color. We demonstrate 18 the OptoArm’s power in a population-based study on contributions of motor circuit cells to age-related motility 19 decline. -
Spotlight on Pain: Optogenetic Approaches for Interrogating Somatosensory Circuits Bryan A
Topical Review Spotlight on pain: optogenetic approaches for interrogating somatosensory circuits Bryan A. Copits, Melanie Y. Pullen, Robert W. Gereau IV* 1. Introduction long-lasting changes in activity.9,16 Recently, using the crystal 74 The implementation of optogenetic tools to manipulate structure of channelrhodopsin, rationale-based protein engi- neering strategies transformed excitatory opsins into chloride neuronal activity with light is transforming our ability to 13,14,155,157 investigate neural systems. By expressing light-sensitive channels for prolonged optical inhibition. proteins, or opsins, in genetically defined neuronal popula- In addition to rapid control of ion flux, molecular engineering tions, optogenetic approaches permit new experimental strategies have developed optical approaches for manipulat- questions that span from the specific properties of defined ing intracellular signaling cascades of cell-surface G-protein- synaptic connections to their roles in complex behav- coupled receptors (GPCRs). Endowing light sensitivity to iors.41,57,106,121 In this review, we focus on opsins for GPCRs was achieved by splicing the intracellular loops of GPCRs of interest to the extracellular and transmembrane manipulating neuronal activity and discuss their biophysical 3,75 properties, delivery strategies, and how these techniques have domains of the light-sensitive GPCR rhodopsin. This been adapted to unravel somatosensory circuits. chimeric approach has generated photoactivatable adrener- gic,3,75,138 serotonin,116 m-opioid,10,137 dopamine,59 adeno- sine,86 and metabotropic glutamate receptors.159 Different 2. Opsin features optoXRs can couple to their endogenous G-protein-mediated Light-gated ion flux was first identified in extremophile intracellular signaling cascades to modulate second messen- 114,115 Fig. -
Genetically Encoded Calcium Indicators for Multi-Color Neural Activity Imaging and Combination with Optogenetics
ORIGINAL RESEARCH ARTICLE published: 04 March 2013 MOLECULAR NEUROSCIENCE doi: 10.3389/fnmol.2013.00002 Genetically encoded calcium indicators for multi-color neural activity imaging and combination with optogenetics Jasper Akerboom 1, Nicole Carreras Calderón 1,2,3, Lin Tian 1†, Sebastian Wabnig 4, Matthias Prigge 5, Johan Tolö 6, Andrew Gordus 7, Michael B. Orger 8,9, Kristen E. Severi 8, John J. Macklin 1, Ronak Patel 1, Stefan R. Pulver 1, Trevor J. Wardill 1†, Elisabeth Fischer 4, Christina Schüler 4,Tsai-WenChen1, Karen S. Sarkisyan 1, Jonathan S. Marvin 1, Cornelia I. Bargmann 7, Douglas S. Kim 1, Sebastian Kügler 6, Leon Lagnado 2, Peter Hegemann 5, Alexander Gottschalk 4, Eric R. Schreiter 1,3 and Loren L. Looger1* 1 Janelia Farm Research Campus, Howard Hughes Medical Institute, Ashburn, VA, USA 2 Medical Research Council Laboratory of Molecular Biology, Cambridge, UK 3 Department of Chemistry, University of Puerto Rico - Río Piedras, San Juan, PR, USA 4 Institute of Biochemistry and Buchmann Institute for Molecular Life Sciences, Johann Wolfgang Goethe-University Frankfurt, Frankfurt, Germany 5 Experimentelle Biophysik, Humboldt Universität zu Berlin, Berlin, Germany 6 Department of Neurology, University Medicine Göttingen, Göttingen, Germany 7 Laboratory of Neural Circuits and Behavior, Howard Hughes Medical Institute, The Rockefeller University, New York, NY, USA 8 Department of Molecular and Cellular Biology, Center for Brain Science, Harvard University, Cambridge, MA, USA 9 Champalimaud Neuroscience Programme, Champalimaud Centre for the Unknown, Lisboa, Portugal Edited by: Genetically encoded calcium indicators (GECIs) are powerful tools for systems Piotr Bregestovski, Institut national neuroscience. Here we describe red, single-wavelength GECIs, “RCaMPs,” engineered de la santé et de la recherche from circular permutation of the thermostable red fluorescent protein mRuby. -
Evolution of the Bacteriorhodopsin Gene Bop in Haloarchaea Maulik Jitesh Jani University of Connecticut - Storrs, [email protected]
University of Connecticut OpenCommons@UConn Honors Scholar Theses Honors Scholar Program Spring 5-6-2012 Evolution of the bacteriorhodopsin gene bop in haloarchaea Maulik Jitesh Jani University of Connecticut - Storrs, [email protected] Follow this and additional works at: https://opencommons.uconn.edu/srhonors_theses Part of the Biology Commons, and the Other Ecology and Evolutionary Biology Commons Recommended Citation Jani, Maulik Jitesh, "Evolution of the bacteriorhodopsin gene bop in haloarchaea" (2012). Honors Scholar Theses. 268. https://opencommons.uconn.edu/srhonors_theses/268 Jani 1 Evolution of the bacteriorhodopsin gene bop in haloarchaea Maulik J. Jani Jani 2 Evolution of the bacteriorhodopsin gene bop in haloarchaea Maulik Jani, R. Thane Papke Summary: Rhodopsins are among the most studied protein families. They all incorporate a light based chromophore and an opsin. They are found in all three domains of life and can be found in diverse environments, such as the membrane of the haloarchaea, Haloarcula (Haa) marismortui, growing on salt flats in Death Valley, to within human tissues and deep sea water (Briggs and Spudich 2005). Rhodopsins can serve multifarious purposes, from phototaxis away from harmful light to efficient energy generation. Bacteriorhodopsin is the best studied rhodopsin in haloarchaea. The evolutionary pattern of bacteriorhodopsin through its gene, bop, is the main focus of this study. Planned as a comparative study of bop against expected phylogeny constructed on 16S rRNA gene sequences, as well as phylogeny based on a tree constructed from core genes; this study also hoped to find possible examples of Horizontal Gene Transfer (HGT). The bop gene was amplified and sequenced from multiple strains, and additional sequences were gathered from the National Center for Biotechnology Information (NCBI), bringing a total of 51 sequences from 41 strains covering 15 recognized genera.