Trans-Β-Galactosidase Activity of Pig Enzymes Embedded in the Small
Total Page:16
File Type:pdf, Size:1020Kb
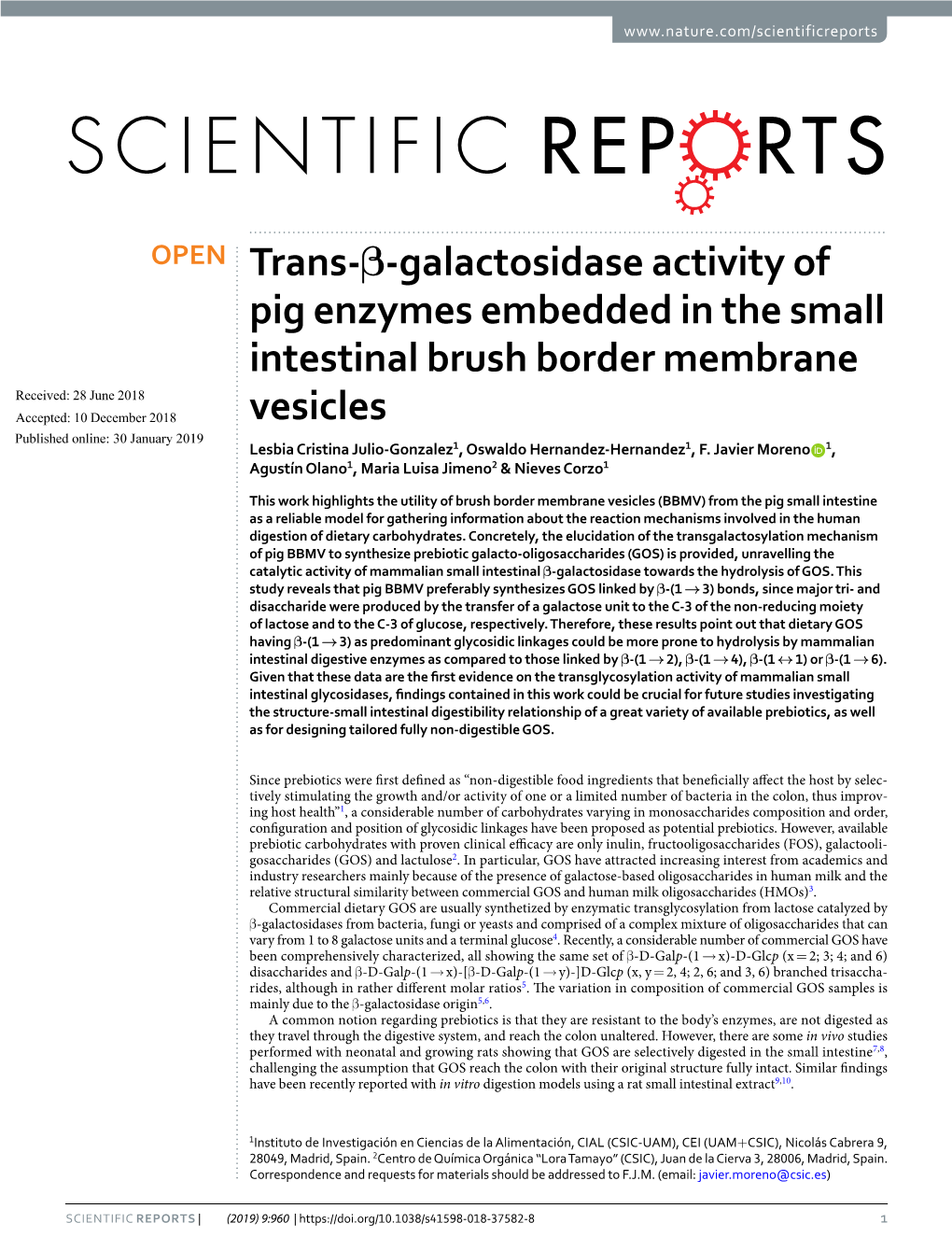
Load more
Recommended publications
-
METACYC ID Description A0AR23 GO:0004842 (Ubiquitin-Protein Ligase
Electronic Supplementary Material (ESI) for Integrative Biology This journal is © The Royal Society of Chemistry 2012 Heat Stress Responsive Zostera marina Genes, Southern Population (α=0. -
Increasing the Amylose Content of Durum Wheat Through Silencing of the Sbeiia Genes
Sestili et al. BMC Plant Biology 2010, 10:144 http://www.biomedcentral.com/1471-2229/10/144 RESEARCH ARTICLE Open Access IncreasingResearch article the amylose content of durum wheat through silencing of the SBEIIa genes Francesco Sestili1, Michela Janni1, Angela Doherty2, Ermelinda Botticella1, Renato D'Ovidio1, Stefania Masci1, Huw D Jones2 and Domenico Lafiandra*1 Abstract Background: High amylose starch has attracted particular interest because of its correlation with the amount of Resistant Starch (RS) in food. RS plays a role similar to fibre with beneficial effects for human health, providing protection from several diseases such as colon cancer, diabetes, obesity, osteoporosis and cardiovascular diseases. Amylose content can be modified by a targeted manipulation of the starch biosynthetic pathway. In particular, the inactivation of the enzymes involved in amylopectin synthesis can lead to the increase of amylose content. In this work, genes encoding starch branching enzymes of class II (SBEIIa) were silenced using the RNA interference (RNAi) technique in two cultivars of durum wheat, using two different methods of transformation (biolistic and Agrobacterium). Expression of RNAi transcripts was targeted to the seed endosperm using a tissue-specific promoter. Results: Amylose content was markedly increased in the durum wheat transgenic lines exhibiting SBEIIa gene silencing. Moreover the starch granules in these lines were deformed, possessing an irregular and deflated shape and being smaller than those present in the untransformed controls. Two novel granule bound proteins, identified by SDS- PAGE in SBEIIa RNAi lines, were investigated by mass spectrometry and shown to have strong homologies to the waxy proteins. RVA analysis showed new pasting properties associated with high amylose lines in comparison with untransformed controls. -
Product Guide
www.megazyme.com es at dr hy o rb a C • s e t a r t s b u S e m y z n E • s e m y z n E • s t i K y a s s A Plant Cell Wall & Biofuels Product Guide 1 Megazyme Test Kits and Reagents Purity. Quality. Innovation. Barry V. McCleary, PhD, DScAgr Innovative test methods with exceptional technical support and customer service. The Megazyme Promise. Megazyme was founded in 1988 with the We demonstrate this through the services specific aim of developing and supplying we offer, above and beyond the products we innovative test kits and reagents for supply. We offer worldwide express delivery the cereals, food, feed and fermentation on all our shipments. In general, technical industries. There is a clear need for good, queries are answered within 48 hours. To validated methods for the measurement of make information immediately available to the polysaccharides and enzymes that affect our customers, we established a website in the quality of plant products from the farm 1994, and this is continually updated. Today, it gate to the final food. acts as the source of a wealth of information on Megazyme products, but also is the hub The commitment of Megazyme to “Setting of our commercial activities. It offers the New Standards in Test Technology” has been possibility to purchase and pay on-line, to continually recognised over the years, with view order history, to track shipments, and Megazyme and myself receiving a number many other features to support customer of business and scientific awards. -
Studies of Barley Limit Dextrinase I I
b ¡\il i: ilq.5l¡Tt-!iÈ' ta. S.S LIBRARY STUDIES OF BARLEY LIMIT DEXTRINASE I I I I by I l I ¡ I Michael John Sissons ì B.Ag.Sc. (University of Adelaide)' I I M.Ag.Sc. (La Trobe UniversitY) i l A thesis submitted to the University of Adelaide for the degree of Doctor of Philosophy Departnent of Plant Science, Waite Agricultural Resea¡ch Institute, Glen Osmond, South Australia October, 1991 LIST OF CONTENTS Contents Page No. Summary i-ü Statement of originality and consent for photocopy or loan lU Acknowledgements iv List of publications v Chapter 1: LITERATURE REVIEW 1.1 Introduction I 1.2 Properties of limit dextrinase 2 t.2.r Purification 2 1.2.2 Enzyme properties 3 t.2.3 Effect of inhibitors 3 r.2.4 Polymorphism 7 t.2.5 Substrate specificity 7 1.2.5.1 Action of limit dextrinase on oligosaccharides 7 t.2.5.2 Polysaccharides 7 1.3 Methods of Assay 9 1.3.1 Detection of Limit Dextrinase Activity in Electrophoretic Gels 10 t.4 Synthesis of limit dextrinase t2 1.4.1 Mechanism of Increase in Limit Dextrinase Activity during Germination 13 1.5 Effect of Barley Genotype and Environment on Limit Dextrinase ActivitY 15 1.6 Limit dextrinase - Role in Malting and Brewing 16 1.6.1 Effect of Kilning on Limit Dextrinase Activity 18 r.6.2 Effect of Mashing on Limit Dextrinase Activity 18 1.6.3 Limit 0extrinase-Role in Speciality Brewing, Distiling and Related Iridustries 2l 1.6.4 Relationship beween Limit Dextrinase Activity, Wort Fermentability and Alcohol Production 22 r.7 Role of Limit Dextrinase in Starch Degradation 23 1.8 Conclusions 24 Chapter -
Evaluation of Saccharifying Methods for Alcoholic Fermentation of Starchy Substrates Alice Lee Iowa State College
Iowa State University Capstones, Theses and Retrospective Theses and Dissertations Dissertations 1955 Evaluation of saccharifying methods for alcoholic fermentation of starchy substrates Alice Lee Iowa State College Follow this and additional works at: https://lib.dr.iastate.edu/rtd Part of the Biochemistry Commons Recommended Citation Lee, Alice, "Evaluation of saccharifying methods for alcoholic fermentation of starchy substrates " (1955). Retrospective Theses and Dissertations. 13626. https://lib.dr.iastate.edu/rtd/13626 This Dissertation is brought to you for free and open access by the Iowa State University Capstones, Theses and Dissertations at Iowa State University Digital Repository. It has been accepted for inclusion in Retrospective Theses and Dissertations by an authorized administrator of Iowa State University Digital Repository. For more information, please contact [email protected]. NOTE TO USERS This reproduction is the best copy available. ® UMI EVALUATION OF SACCHARIFYING METHODS FOR ALCOHOLIC FERMENTATION OF STARCHY SUBSTRATES by Alice Lee A Dlseertatlon Submitted to the Graduate Faculty In Partial Fulfillment of The RequirementB for the Degree of DOCTOR OF PHILOSOPHY Major Subjects Blochensletry Approved: Signature was redacted for privacy. In Charge of Major Work Signature was redacted for privacy. Head of Major Department Signature was redacted for privacy. Dean of Graduate College Iowa State College 1955 UMI Number: DP12815 INFORMATION TO USERS The quality of this reproduction is dependent upon the quality of the copy submitted. Broken or indistinct print, colored or poor quality illustrations and photographs, print bleed-through, substandard margins, and improper alignment can adversely affect reproduction. In the unlikely event that the author did not send a complete manuscript and there are missing pages, these will be noted. -
STUDY of STARCH DEBRANCHING ENZYMES in DEVELOPING WHEAT KERNELS a Thesis Submitted to the College of Graduate Studies and Resear
STUDY OF STARCH DEBRANCHING ENZYMES IN DEVELOPING WHEAT KERNELS A Thesis Submitted to the College of Graduate Studies and Research in Partial Fulfilment of the Requirements for the Degree of Doctor of Philosophy in the Department of Biochemistry University of Saskatchewan Saskatoon By Supatcharee Netrphan Spring 2002 © Copyright Supatcharee Netrphan, 2002. All rights reserved. PERMISSION TO USE In presenting this thesis in partial fulfilment of the requirements for a Postgraduate degree from the University of Saskatchewan, I agree that the Libraries of this University may make it freely available for inspection. I further agree that permission for copying of this thesis in any manner, in whole or in part, for scholarly purposes may be granted by the professor or professors who supervised my thesis work or, in their absence, by the Head of the Department or the Dean of the College in which my thesis work was done. It is understood that any copying or publication or use of this thesis or parts thereof for financial gain shall not be allowed without my written permission. It is also understood that due recognition shall be given to me and to the University of Saskatchewan in any scholarly use which may be made of any material in my thesis. Requests for permission to copy or to make other use of material in this thesis in whole or in part should be addressed to: Head of the Department of Biochemistry University of Saskatchewan 107 Wiggins Road Saskatoon, Saskatchewan S7N 5E5 i ABSTRACT Starch debranching enzymes, which specifically hydrolyse a-1,6 glucosidic bonds in glucans containing both a-1,4 and a-1,6 linkages, are classified into two types: isoamylase (EC. -
Source: the Arabidopsis Information Resource (TAIR);
Table S1 List of targeted loci and information about their function in Arabidopsis thaliana (source: The Arabidopsis Information Resource (TAIR); https://www.arabidopsis.org/tools/bulk/genes/index.jsp). Locus Gene Model Gene Model Description Gene Model Primary Gene Symbol All Gene Symbols Identifier Name Type AT1G78800 AT1G78800.1 UDP-Glycosyltransferase superfamily protein_coding protein;(source:Araport11) AT5G06830 AT5G06830.1 hypothetical protein;(source:Araport11) protein_coding AT2G31740 AT2G31740.1 S-adenosyl-L-methionine-dependent methyltransferases protein_coding superfamily protein;(source:Araport11) AT5G11960 AT5G11960.1 magnesium transporter, putative protein_coding (DUF803);(source:Araport11) AT4G00560 AT4G00560.4 NAD(P)-binding Rossmann-fold superfamily protein_coding protein;(source:Araport11) AT1G80510 AT1G80510.1 Encodes a close relative of the amino acid transporter ANT1 protein_coding (AT3G11900). AT2G21250 AT2G21250.1 NAD(P)-linked oxidoreductase superfamily protein_coding protein;(source:Araport11) AT5G04420 AT5G04420.1 Galactose oxidase/kelch repeat superfamily protein_coding protein;(source:Araport11) AT4G34910 AT4G34910.1 P-loop containing nucleoside triphosphate hydrolases protein_coding superfamily protein;(source:Araport11) AT5G66120 AT5G66120.2 3-dehydroquinate synthase;(source:Araport11) protein_coding AT1G45110 AT1G45110.1 Tetrapyrrole (Corrin/Porphyrin) protein_coding Methylase;(source:Araport11) AT1G67420 AT1G67420.2 Zn-dependent exopeptidases superfamily protein_coding protein;(source:Araport11) AT3G62370 -
Structure of the Starch-Debranching Enzyme Barley Limit Dextrinase Reveals Homology of the N-Terminal Domain to CBM21
View metadata,Downloaded citation and from similar orbit.dtu.dk papers on:at core.ac.uk Dec 20, 2017 brought to you by CORE provided by Online Research Database In Technology Structure of the starch-debranching enzyme barley limit dextrinase reveals homology of the N-terminal domain to CBM21 Møller, Marie Sofie; Abou Hachem, Maher ; Svensson, Birte; Henriksen, Anette Published in: Acta Crystallographica. Section F: Structural Biology and Crystallization Communications Online Link to article, DOI: 10.1107/S1744309112031004 Publication date: 2012 Document Version Publisher's PDF, also known as Version of record Link back to DTU Orbit Citation (APA): Møller, M. S., Abou Hachem, M., Svensson, B., & Henriksen, A. (2012). Structure of the starch-debranching enzyme barley limit dextrinase reveals homology of the N-terminal domain to CBM21. Acta Crystallographica. Section F: Structural Biology and Crystallization Communications Online, 68(Pt 9), 1008-1012. DOI: 10.1107/S1744309112031004 General rights Copyright and moral rights for the publications made accessible in the public portal are retained by the authors and/or other copyright owners and it is a condition of accessing publications that users recognise and abide by the legal requirements associated with these rights. • Users may download and print one copy of any publication from the public portal for the purpose of private study or research. • You may not further distribute the material or use it for any profit-making activity or commercial gain • You may freely distribute the URL identifying the publication in the public portal If you believe that this document breaches copyright please contact us providing details, and we will remove access to the work immediately and investigate your claim. -
Enzymes and Beermaking Streamlining Enzyme Analysis in Brewing
Enzymes and Beermaking Streamlining Enzyme Analysis in Brewing www.megazyme.com CONTENTS Research is at the core of Megazyme’s product development. Continual innovation has allowed us to introduce advances and improvements to accepted, industry-standard methods of analysis. Megazyme products offer: • reduced reaction times • unrivalled enzyme purity • improved enzyme stability (resulting in a longer ‘shelf-life’) • novel assays with ‘best-in-class’ selectivity for the analyte in question • extended cofactor stability (offered in a stable tablet form or as improved liquid formulations with extended expiry dates) Megazyme test kits - researched and manufactured in-house - have attracted worldwide acclaim for their novel methodologies and for the exceptional purity of their enzymes. Streamlining Enzyme Analysis in Brewing 3 • The traditional approach to malt and beer analysis • Enzymes and beer • Measuring enzyme activity • Starch hydrolases: a-Amylase, b-Amylase and Limit-Dextrinase • Cell wall hydrolases: Malt b-Glucanase, Xylanase and Cellulase Assay Kits for the Measurement of Enzyme Activity in Brewing 9 • Starch hydrolases: a-Amylase, b-Amylase, Malt Amylase and Limit-Dextrinase/Pullulanase • Cell wall hydrolases: b-Glucanase, Xylanase and Cellulase Analytical Solutions from Megazyme 11 • Cultivating excellence in cereal chemistry • Tailor-made substrates • Megazyme assays explained STREAMLINING ENZYME ANALYSIS IN BREWING DR. CLAUDIO CORNAGGIA IN THEIR EFFORTS TO INNOVATE IN A DYNAMIC MARKET, BREWERS AIM TO ACHIEVE THE EFFICIENT PRODUCTION OF BETTER BEERS WITH MORE CONSISTENT SENSORY PROPERTIES AT A REDUCED COST. Technical advancements have been applied Brewers are typically comfortable with the methods to all stages of beer production, from cereal they trained to use at the start of their careers, and are harvesting to filtration to bottling and packaging. -
Supplementary Information
Supplementary Information Supplementary Figure 1: Size exclusion chromatography of 2G12. .......................................................... 2 Supplementary Figure 2: SDS-PAGE of SEC purified 2G12. ..................................................................... 3 Supplementary Figure 3: Native mass spectrometry of 2G12 and contrast calibration. ............................. 4 Supplementary Figure 4: Dynamic range estimation, sample carriers and diffusion correction. ................ 5 Supplementary Figure 5: Data analysis approaches for 2G12 monomer/dimer ratios using sample gaskets. .................................................................................................................................................... 6 Supplementary Figure 6: Technical replicates of purity screening of trastuzumab and FcγRIa. ................ 7 Supplementary Figure 7: Technical replicates of IgG-FcγIa binding. ......................................................... 8 Supplementary Figure 8: Native MS to confirm IgG deglycosylation. ........................................................ 9 Supplementary Figure 10: Screening method for quantifying binding affinities and kinetics with MP. ..... 11 Supplementary Figure 11: Concentration-dependent Kd distribution for IgGdeglycosylated-FcγIa. ................. 12 Supplementary Figure 12: Equilibration time screening and assignment of FcγRIa molecular mass. ..... 13 Supplementary Figure 13: Equilibration time screening of Kd for IgGdeglycosylated-FcγIa. ........................... -
Barley Malt Limit Dextrinase: Varietal, Environmental, and Malting Effects12
Barley Malt Limit Dextrinase: Varietal, Environmental, and Malting Effects12 W. J. Lee and R. E. Pyler,3 Department of Cereal Chemistry and Technology, North Dakota State University, Fargo 58105 ABSTRACT oligosaccharides, producing new reducing groups. After this initial digestion, the reaction mixture is heated to A new assay procedure was used to study the limit dextrinase activity of destroy all enzyme activity, and an aliquot is treated with a- barley malt. Variety, location, and crop year were shown to significantly glucosidase to convert maltotriose, maltose, and other starch- affect the production of limit dextrinase. Enzyme activity remained at a derived substrates to glucose, which we have estimated very low level during steeping and up to the third day of germination but increased substantially after the fifth day. Gibberellic acid had a stimulatory enzymatically. A blank containing no pullulan is run concurrently effect on limit dextrinase activity. No loss in activity occurred during to estimate starch-derived glucose. Thus, this procedure provides kilning at 49° C for 24 hr, but activity declined as kilning temperatures an estimate of limit dextrinase activity, which is unaffected by the increased. In ASBC mashes, 100% of the activity remained after 30 min at presence of a- and /3-amylase and endogenous a-glucosidase. 45° C but began to decline as mash temperatures rose to 70° C. Ground Using this assay procedure, we proceeded to study limit malts containing varying levels of limit dextrinase activity were added to dextrinase with respect to its development during germination, the fermenting wort, and alcohol production and the extent of fermentability of effect on its activity of kiln and mashing heat, its effects on a-limit the wort were closely related to limit dextrinase activity. -
A New Approach to Limit Dextrinase and Its Role in Mashing, Pp.205-210 Volume 105, No
A New Approach to Limit Dextrinase and its Role in Mashing, pp.205-210 Volume 105, No. 4,1999 A New Approach to Limit Dextrinase and its Role in Mashing* by Katharina Stenholm1-2 and Silja Home1 1. VTT Biotechnology and Food Research, PO Box 1500, F1N-02044 VTT, Finland 2. Present address: Oy Lahden Polttimo Ah, PO Box 22, FIN-15141 LAHTl, Finland Received 22nd December 1998 Limit dextrinase (EC 3.1.2.41) is a debranching enzyme catalyzing the hydrolysis of a-1,6- glucosidic linkages in starch. The role of this debranching enzyme in beer brewing has been questioned due to its assumed heat lability. In the present work the effectiveness of limit dextrinase was studied under conditions mimicking brewery practice rather than in a buffer solution. It was demonstrated that typical conversion temperatures of 63-65 °C and a mash pH of 5.4-5.5 favour the action of malt limit dextrinase. The temperature optimum for the limit dextrinase of a malt extract was 60-62.5° C, as opposed to 50°C for purified limit dextrinase. Lowering the mash pH from 5.8 to 5.4 increased wort fermentability due to increased limit dextrinase activity. Wort fermentability was more strongly correlated to the free limit dextrinase activity of malt than to the a- and fi-amylase activities. Key Words: Limit dextrinase, dextrins, mashing, fermentability. INTRODUCTION The aim of the present work was to investigate the Starch consists of essentially unbranched amylose and properties of malt limit dextrinase that influence its highly branched amylopectin. In mashing, amylose is effectiveness in mashing and to evaluate the importance rapidly and extensively degraded by a-amylase and [}- of limit dextrinase in brewing.