University of Florida Thesis Or
Total Page:16
File Type:pdf, Size:1020Kb
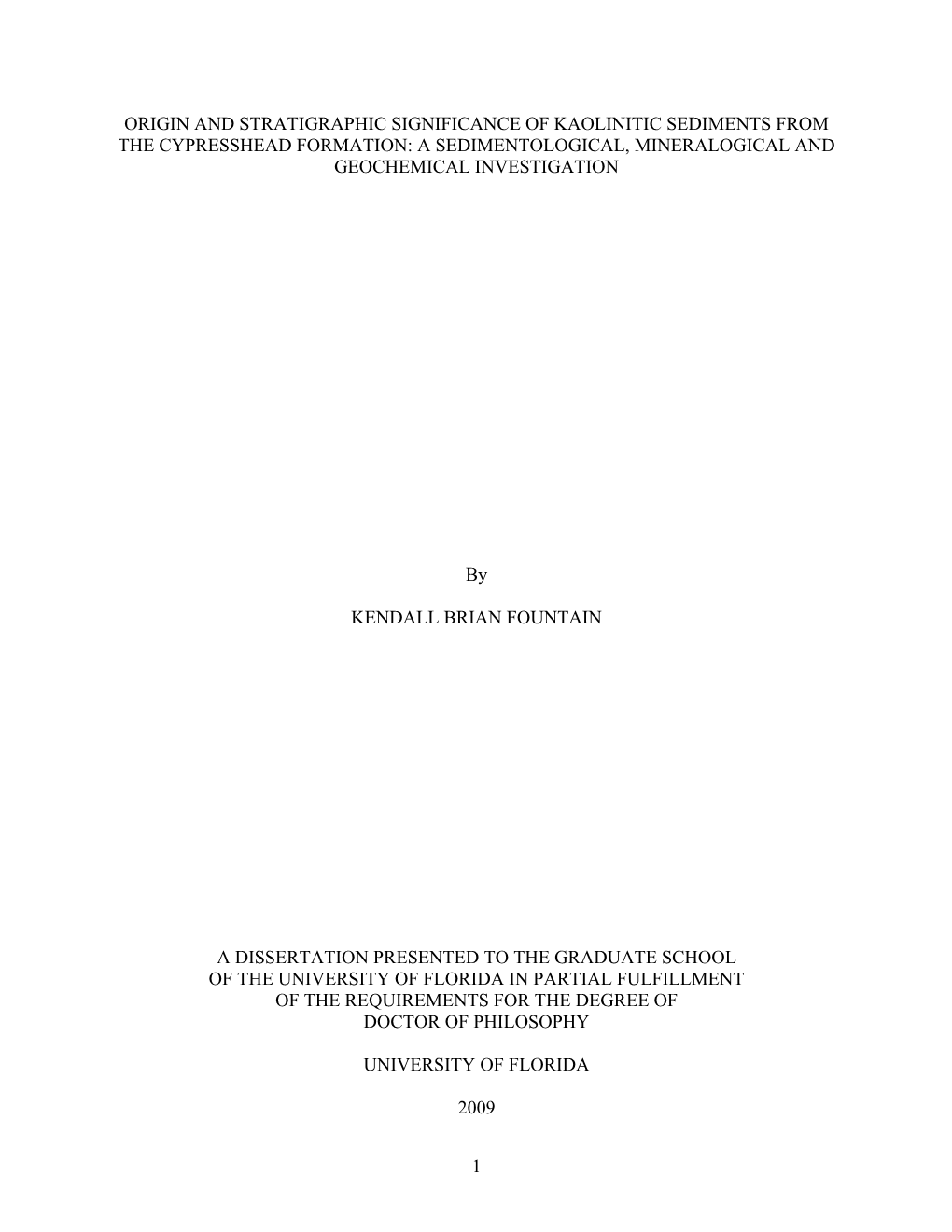
Load more
Recommended publications
-
State of Florida Department Of
STATE OF FLORIDA DEPARTMENT OF ENVIRONMENTAL PROTECTION Herschel T. Vinyard Jr., Secretary REGULATORY PROGRAMS Jeff Littlejohn, Deputy Secretary FLORIDA GEOLOGICAL SURVEY Jonathan D. Arthur, State Geologist and Director OPEN-FILE REPORT 97 Text to accompany geologic map of the western portion of the USGS Inverness 30 x 60 minute quadrangle, central Florida By Christopher P. Williams and Richard C. Green 2012 ISSN (1058-1391) This geologic map was funded in part by the USGS National Cooperative Geologic Mapping Program under assistance award number G11AC20418 in Federal fiscal year 2011 TABLE OF CONTENTS ABSTRACT .................................................................................................................................... 1 INTRODUCTION .......................................................................................................................... 1 Methods .......................................................................................................................................... 3 Previous Work ................................................................................................................................ 5 GEOLOGIC SUMMARY .............................................................................................................. 5 Structure .......................................................................................................................................... 7 Geomorphology ........................................................................................................................... -
Exhibit Specimen List FLORIDA SUBMERGED the Cretaceous, Paleocene, and Eocene (145 to 34 Million Years Ago) PARADISE ISLAND
Exhibit Specimen List FLORIDA SUBMERGED The Cretaceous, Paleocene, and Eocene (145 to 34 million years ago) FLORIDA FORMATIONS Avon Park Formation, Dolostone from Eocene time; Citrus County, Florida; with echinoid sand dollar fossil (Periarchus lyelli); specimen from Florida Geological Survey Avon Park Formation, Limestone from Eocene time; Citrus County, Florida; with organic layers containing seagrass remains from formation in shallow marine environment; specimen from Florida Geological Survey Ocala Limestone (Upper), Limestone from Eocene time; Jackson County, Florida; with foraminifera; specimen from Florida Geological Survey Ocala Limestone (Lower), Limestone from Eocene time; Citrus County, Florida; specimens from Tanner Collection OTHER Anhydrite, Evaporite from early Cenozoic time; Unknown location, Florida; from subsurface core, showing evaporite sequence, older than Avon Park Formation; specimen from Florida Geological Survey FOSSILS Tethyan Gastropod Fossil, (Velates floridanus); In Ocala Limestone from Eocene time; Barge Canal spoil island, Levy County, Florida; specimen from Tanner Collection Echinoid Sea Biscuit Fossils, (Eupatagus antillarum); In Ocala Limestone from Eocene time; Barge Canal spoil island, Levy County, Florida; specimens from Tanner Collection Echinoid Sea Biscuit Fossils, (Eupatagus antillarum); In Ocala Limestone from Eocene time; Mouth of Withlacoochee River, Levy County, Florida; specimens from John Sacha Collection PARADISE ISLAND The Oligocene (34 to 23 million years ago) FLORIDA FORMATIONS Suwannee -
Subsurface Geology of Cenozoic Deposits, Gulf Coastal Plain, South-Central United States
REGIONAL STRATIGRAPHY AND _^ SUBSURFACE GEOLOGY OF CENOZOIC DEPOSITS, GULF COASTAL PLAIN, SOUTH-CENTRAL UNITED STATES V U.S. GEOLOGICAL SURVEY PROFESSIONAL PAPER 1416-G AVAILABILITY OF BOOKS AND MAPS OF THE U.S. GEOLOGICAL SURVEY Instructions on ordering publications of the U.S. Geological Survey, along with prices of the last offerings, are given in the current-year issues of the monthly catalog "New Publications of the U.S. Geological Survey." Prices of available U.S. Geological Survey publications re leased prior to the current year are listed in the most recent annual "Price and Availability List." Publications that may be listed in various U.S. Geological Survey catalogs (see back inside cover) but not listed in the most recent annual "Price and Availability List" may no longer be available. Reports released through the NTIS may be obtained by writing to the National Technical Information Service, U.S. Department of Commerce, Springfield, VA 22161; please include NTIS report number with inquiry. Order U.S. Geological Survey publications by mail or over the counter from the offices listed below. BY MAIL OVER THE COUNTER Books Books and Maps Professional Papers, Bulletins, Water-Supply Papers, Tech Books and maps of the U.S. Geological Survey are available niques of Water-Resources Investigations, Circulars, publications over the counter at the following U.S. Geological Survey offices, all of general interest (such as leaflets, pamphlets, booklets), single of which are authorized agents of the Superintendent of Docu copies of Earthquakes & Volcanoes, Preliminary Determination of ments. Epicenters, and some miscellaneous reports, including some of the foregoing series that have gone out of print at the Superintendent of Documents, are obtainable by mail from ANCHORAGE, Alaska-Rm. -
Tulane Studies Tn Geology and Paleontology Pliocene
TULANE STUDIES TN GEOLOGY AND PALEONTOLOGY Volu me 22, Number 2 Sepl<'mber 20. l!J8~) PLIOCENE THREE-TOED HORSES FROM LOUISIANA. WITH COMMENTS ON THE CITRONELLE FORMATION EAHL M. MANNING MUSP.UM OF'GEOSCIF:NCE. LOUISJJ\NA STATE UNIVF:RSlTY. JJATO.\I ROI.JG/<. LOL'/S//\;\':1 and llRUCE J. MACFADDlrn DEJ>ARTM/<:NTOF NATUH/\LSCIENCES. F'LORJD/\ MUSf:UM Of<'NJ\TUIV\/, lllSTOUY UNIVERSITY OF FLOH!IJJ\. GJ\/NESVlU.E. Fl.OH/DA CONTENTS Page T. ABSTRACT 3.5 II INTRODUCTION :l5 Ill. ACKNOWLEDGMENTS :rn TV . ABBREVIATIONS :l7 V. SYSTEMATIC PALEONTOLOGY ;37 VI. AGE OF THE TUNICA HILLS HIPPARIONINES 38 VIL STRATIGRAPHIC PROVENIENCE 38 Vlll. PLIOCENE TERRESTRIAL VERTEBRATES OF THE GULF AND ATLANTIC COASTAL PLAIN .JO IX. COMMENTS ON THE CITRONELLE FORMATION .JI X. AGE OF THE CITRONELLE 42 XL TH E CITRONELLE FORMATION IN nm TUNICA HILLS .t:1 XII. LITERATURE CITED l.J January of 1985, the senior author was L ABSTRACT shown a large collection of late Pleistocene Teeth and metacarpals of early Pliocene (Rancholabrean land-mammal agel ver (latest Hemphillian land-mammal age) tebrate fossils from the Tunica Hills of three-toed (hipparionine) horses are de Louisiana (Fig. I) by Dr. A. Bradley scribed from the Tunica Hills of West McPherson of Centenary College, Feliciana Parish in east-central Louisiana. Shreveport. McPherson and Mr. Bill Lee An upper molar perta ins to Nannippus of Balon Rouge had collected fossils from minor, known from the Hcmphillian of that area since about 1981. Among the Central and North America, and two teeth standard assemblage of Rancholabrean and two distal metacarpals pertain to a re taxa (e.g. -
Systematics, Climate, and Ecology of Fossil and Extant Nyssa (Nyssaceae, Cornales) and Implications of Nyssa Grayensis Sp
East Tennessee State University Digital Commons @ East Tennessee State University Electronic Theses and Dissertations Student Works 8-2013 Systematics, Climate, and Ecology of Fossil and Extant Nyssa (Nyssaceae, Cornales) and Implications of Nyssa grayensis sp. nov. from the Gray Fossil Site, Northeast Tennessee Nathan R. Noll East Tennessee State University Follow this and additional works at: https://dc.etsu.edu/etd Part of the Biodiversity Commons, Climate Commons, Paleontology Commons, and the Plant Biology Commons Recommended Citation Noll, Nathan R., "Systematics, Climate, and Ecology of Fossil and Extant Nyssa (Nyssaceae, Cornales) and Implications of Nyssa grayensis sp. nov. from the Gray Fossil Site, Northeast Tennessee" (2013). Electronic Theses and Dissertations. Paper 1204. https://dc.etsu.edu/etd/1204 This Thesis - Open Access is brought to you for free and open access by the Student Works at Digital Commons @ East Tennessee State University. It has been accepted for inclusion in Electronic Theses and Dissertations by an authorized administrator of Digital Commons @ East Tennessee State University. For more information, please contact [email protected]. Systematics, Climate, and Ecology of Fossil and Extant Nyssa (Nyssaceae, Cornales) and Implications of Nyssa grayensis sp. nov. from the Gray Fossil Site, Northeast Tennessee ___________________________ A thesis presented to the faculty of the Department of Biological Sciences East Tennessee State University In partial fulfillment of the requirements for the degree Master of Science in Biology ___________________________ by Nathan R. Noll August 2013 ___________________________ Dr. Yu-Sheng (Christopher) Liu, Chair Dr. Tim McDowell Dr. Foster Levy Keywords: Nyssa, Endocarp, Gray Fossil Site, Miocene, Pliocene, Karst ABSTRACT Systematics, Climate, and Ecology of Fossil and Extant Nyssa (Nyssaceae, Cornales) and Implications of Nyssa grayensis sp. -
Biostratigraphy and Diversity Patterns of Cenozoic Echinoderms from Florida
BIOSTRATIGRAPHY AND DIVERSITY PATTERNS OF CENOZOIC ECHINODERMS FROM FLORIDA By CRAIG W. OYEN A DISSERTATION PRESENTED TO THE GRADUATE SCHOOL OF THE UNIVERSITY OF FLORIDA IN PARTIAL FULFILLMENT OF THE REQUIREMENTS FOR THE DEGREE OF DOCTOR OF PHILOSOPHY UNIVERSITY OF FLORIDA 2001 Walter and Norma Oyen, who have always I dedicate this to my parents, expressed absolute confidence in my ability to succeed and supported all my this without their endeavors without question or hesitation. I could not have done it realize. support through all these years and I appreciate more than they ACKNOWLEDGMENTS This project could not have been completed without the aid of many people. Most important is the assistance and direction given by my dissertation committee chair, Dr. Douglas S. Jones. He encouraged me to work on whatever suggestions to improve my topic(s) I found interesting, and simply gave me approach in order to answer any of those questions. He also made my time in Gainesville enjoyable academically and socially by introducing me to other faculty and students, inviting me to his home or restaurants for dinners, and participating in pick-up basketball games and intramural games for relaxation. He (along with Roger Portell) accompanied me on many fascinating fieldtrips in Florida and other locations (often in association with GSA meetings) that expanded my scientific and other perspectives greatly. I thank the other members of my dissertation committee, Drs. Randazzo, Hodell, MacFadden, and Mature, for participating in my research and providing guidance whenever I it to had this group of faculty asked for their help. I consider a pleasure have members participating on my committee because they only treated me with respect and they openly provided suggestions they believed would serve me best in the context of completing my research and degree. -
Ochlockonee River & Bay SWIM Plan
Ochlockonee River and Bay Surface Water Improvement and Management Plan September 2017 Program Development Series 17-02 NORTHWEST FLORIDA WATER MANAGEMENT DISTRICT GOVERNING BOARD George Roberts Jerry Pate John Alter Chair, Panama City Vice Chair, Pensacola Secretary-Treasurer, Malone Gus Andrews Jon Costello Marc Dunbar DeFuniak Springs Tallahassee Tallahassee Ted Everett Nick Patronis Bo Spring Chipley Panama City Beach Port St. Joe Brett J. Cyphers Executive Director Headquarters 81 Water Management Drive Havana, Florida 32333-4712 (850) 539-5999 Crestview Econfina Milton 180 E. Redstone Avenue 6418 E. Highway 20 5453 Davisson Road Crestview, Florida 32539 Youngstown, FL 32466 Milton, FL 32583 (850) 683-5044 (850) 722-9919 (850) 626-3101 Ochlockonee River and Bay SWIM Plan Northwest Florida Water Management District Acknowledgements This document was developed by the Northwest Florida Water Management District under the auspices of the Surface Water Improvement and Management (SWIM) Program and in accordance with sections 373.451-459, Florida Statutes. The plan update was prepared under the supervision and oversight of Brett Cyphers, Executive Director and Carlos Herd, Director, Division of Resource Management. Funding support was provided by the National Fish and Wildlife Foundation’s Gulf Environmental Benefit Fund. The assistance and support of the NFWF is gratefully acknowledged. The authors would like to especially recognize members of the public, as well as agency reviewers and staff from the District and from the Ecology and Environment, Inc., team that contributed to the development of this plan. Among those that contributed considerable time and effort to assist in the development of this plan are the following. -
PDF Linkchapter
Index [Italic page numbers indicate major references] Abaco Knoll, 359 116, 304, 310, 323 Bahama Platform, 11, 329, 331, 332, Abathomphalus mayaroensis, 101 Aquia Formation, 97, 98, 124 341, 358, 360 Abbott pluton, 220 aquifers, 463, 464, 466, 468, 471, Bahama volcanic crust, 356 Abenaki Formation, 72, 74, 257, 476, 584 Bahamas basin, 3, 5, 35, 37, 39, 40, 259, 261, 369, 372, 458 Aquitaine, France, 213, 374 50 ablation, 149 Arcadia Formation, 505 Bahamas Fracture Zone, 24, 39, 40, Abyssal Plain, 445 Archaean age, 57 50, 110, 202, 349, 358, 368 Adirondack Mountains, 568 Arctic-North Atlantic rift system, 49, Bahamas Slope, 12 Afar region, Djibouti, 220, 357 50 Bahamas-Cuban Fault system, 50 Africa, 146, 229, 269, 295, 299 Ardsley, New York, 568, 577 Baja California, Mexico, 146 African continental crust, 45, 331, Argana basin, Morocco, 206 Bajocian assemblages, 20, 32 347 Argo Fan, Scotian margin, 279 Balair fault zone, 560 African Craton, 368 Argo Salt Formation, 72, 197, 200, Baltimore Canyon trough, 3, 37, 38, African margin, 45, 374 278, 366, 369, 373 40, 50, 67, 72 , 81, 101, 102, African plate, 19, 39, 44, 49 arkoses, 3 138, 139, 222, 254, 269, 360, Afro-European plate, 197 artesian aquifers, 463 366, 369, 396, 419,437 Agamenticus pluton, 220 Ashley Formation, 126 basement rocks, 74 age, 19, 208, 223 Astrerosoma, 96 carbonate deposits, 79 Agulhas Bank, 146 Atkinson Formation, 116, 117 crustal structure, 4, 5, 46 Aiken Formation, 125 Atlantic basin, 6, 9, 264 faults in, 32 Aikin, South Carolina, 515 marine physiography, 9 geologic -
Miocene Paleontology and Stratigraphy of the Suwannee River Basin of North Florida and South Georgia
MIOCENE PALEONTOLOGY AND STRATIGRAPHY OF THE SUWANNEE RIVER BASIN OF NORTH FLORIDA AND SOUTH GEORGIA SOUTHEASTERN GEOLOGICAL SOCIETY Guidebook Number 30 October 7, 1989 MIOCENE PALEONTOLOGY AND STRATIGRAPHY OF THE SUWANNEE RIVER BASIN OF NORTH FLORIDA AND SOUTH GEORGIA Compiled and edit e d by GARY S . MORGAN GUIDEBOOK NUMBER 30 A Guidebook for the Annual Field Trip of the Southeastern Geological Society October 7, 1989 Published by the Southeastern Geological Society P. 0 . Box 1634 Tallahassee, Florida 32303 TABLE OF CONTENTS Map of field trip area ...... ... ................................... 1 Road log . ....................................... ..... ..... ... .... 2 Preface . .................. ....................................... 4 The lithostratigraphy of the sediments exposed along the Suwannee River in the vicinity of White Springs by Thomas M. scott ........................................... 6 Fossil invertebrates from the banks of the Suwannee River at White Springs, Florida by Roger W. Portell ...... ......................... ......... 14 Miocene vertebrate faunas from the Suwannee River Basin of North Florida and South Georgia by Gary s. Morgan .................................. ........ 2 6 Fossil sirenians from the Suwannee River, Florida and Georgia by Daryl P. Damning . .................................... .... 54 1 HAMIL TON CO. MAP OF FIELD TRIP AREA 2 ROAD LOG Total Mileage from Reference Points Mileage Last Point 0.0 0.0 Begin at Holiday Inn, Lake City, intersection of I-75 and US 90. 7.3 7.3 Pass under I-10. 12 . 6 5.3 Turn right (east) on SR 136. 15.8 3 . 2 SR 136 Bridge over Suwannee River. 16.0 0.2 Turn left (west) on us 41. 19 . 5 3 . 5 Turn right (northeast) on CR 137. 23.1 3.6 On right-main office of Occidental Chemical Corporation. -
Pleistocene Sediment and Vertebrate Fossil Associations in the Mississippi Black Belt: a Genetic Approach
Louisiana State University LSU Digital Commons LSU Historical Dissertations and Theses Graduate School 1974 Pleistocene Sediment and Vertebrate Fossil Associations in the Mississippi Black Belt: a Genetic Approach. John Morgan Kaye Louisiana State University and Agricultural & Mechanical College Follow this and additional works at: https://digitalcommons.lsu.edu/gradschool_disstheses Recommended Citation Kaye, John Morgan, "Pleistocene Sediment and Vertebrate Fossil Associations in the Mississippi Black Belt: a Genetic Approach." (1974). LSU Historical Dissertations and Theses. 2612. https://digitalcommons.lsu.edu/gradschool_disstheses/2612 This Dissertation is brought to you for free and open access by the Graduate School at LSU Digital Commons. It has been accepted for inclusion in LSU Historical Dissertations and Theses by an authorized administrator of LSU Digital Commons. For more information, please contact [email protected]. INFORMATION TO USERS This material was produced from a microfilm copy of the original document. While the most advanced technological means to photograph and reproduce this document have been used, the quality is heavily dependent upon the quality of the original submitted. The following explanation of techniques is provided to help you understand markings or patterns which may appear on this reproduction. 1. The sign or "target" for pages apparently lacking from the document photographed is "Missing Page(s)". If it was possible to obtain the missing page(s) or section, they are spliced into the film along with adjacent pages. This may have necessitated cutting thru an image and duplicating adjacent pages to insure you complete continuity. 2. When an image on the film is obliterated with a large round black mark, it is an indication that the photographer suspected that the copy may have moved during exposure and thus cause a blurred image. -
Chapter 3. Origin and Evolution of Tampa Bay 37
Chapter 3. Origin and Evolution of Tampa Bay 37 Chapter 3. Origin and Evolution of Tampa Bay By Gerold Morrison (AMEC-BCI) and Kimberly K. Yates (U.S. Geological Survey–St. Petersburg, Florida) TAMPA BAY HAS AN UNUSUAL geologic history when compared to many other estuaries in the eastern U.S. (Brooks and Doyle, 1998; Hine and others, 2009). It lies near the center of the carbonate Florida Platform (fig. 3–1), and is associated with a buried “shelf valley system” (including a paleo-channel feature located beneath the modern Egmont Channel) that formed in the early Miocene, about 20 million years ago (Ma) (Hine, 1997; Donahue and others, 2003; Duncan and others, 2003). Since that time the area has been subject to substantial fluctuations in sea level and alternating periods of sediment deposition and removal. These events have produced a complex distribution of siliciclastic and carbonate-based sediments within the bay, its associated barrier islands, and the inner Florida shelf (Brooks and Doyle, 1998; Brooks and others, 2003; Duncan and others, 2003; Ferguson and Davis, 2003). Sinkholes and other karst features in the underlying carbonate strata, which are common throughout the west-central Florida region, have been important factors underlying the development of both Tampa Bay (Brooks and Doyle, 1998; Donahue and others, 2003) and Charlotte Harbor, a geologically similar estuary located about 100 mi to the south (Hine and others, 2009). In the case of Tampa Bay, the underlying shelf valley system consists of multiple karst controlled subbasins (separated by bedrock highs) that have been filled by sediments, some of which were deposited fluvially (Hine and others, 2009). -
Ginsburg IAS Volume
05/02/2007/1420hrs Karst Subbasins and Their Relation to the Transport of Tertiary Siliciclastic Sediments on the Florida Platform Running Title: Karst Subbasins on the Florida Platform ALBERT C. HINE1, *BEAU SUTHARD1, STANLEY D. LOCKER1, KEVIN J. CUNNINGHAM2, DAVID S. DUNCAN3, MARK EVANS4, AND ROBERT A. MORTON5 1 College of Marine Science, University of South Florida, St. Petersburg, FL 33701, [email protected] 2 U.S. Geological Survey,3110 SW 9th Ave, Ft. Lauderdale, FL 33315 3 Department of Marine Science, Eckerd College, 4200 54th Ave So., St. Petersburg, FL 33711 4 Division of Health Assessment and Consultation, NCEH/ATSDR, Mail Stop E-32, 1600 Clifton Rd., Atlanta, GA 30333 5 U.S. Geological Survey, 600 4th St. So., St. Petersburg, FL 33701 *Present Address Coastal Planning and Engineering 2481 NW Boca Raton Blvd Boca Raton, FL 33431 [email protected] 1 ABSTRACT Multiple, spatially-restricted, partly-enclosed karst subbasins with as much as 100 m of relief occur on a mid-carbonate platform setting beneath the modern estuaries of Tampa Bay and Charlotte Harbor located along the west-central Florida coastline. A relatively high-amplitude seismic basement consists of the mostly carbonate, upper Oligocene to middle Miocene Arcadia Formation, which has been significantly deformed into folds, sags, warps and sinkholes. Presumably, this deformation was caused during a mid-to-late Miocene sea-level lowstand by deep-seated dissolution of carbonates, evaporates or both, resulting in collapse of the overlying stratigraphy, thus creating paleotopographic depressions. Seismic sequences containing prograding clinoforms filled approximately 90% of the accommodation space of these western Florida subbasins.