Isotopic Separation and Spectroscopy of Ytterbium-173(3/2) Using Laser Cooling
Total Page:16
File Type:pdf, Size:1020Kb
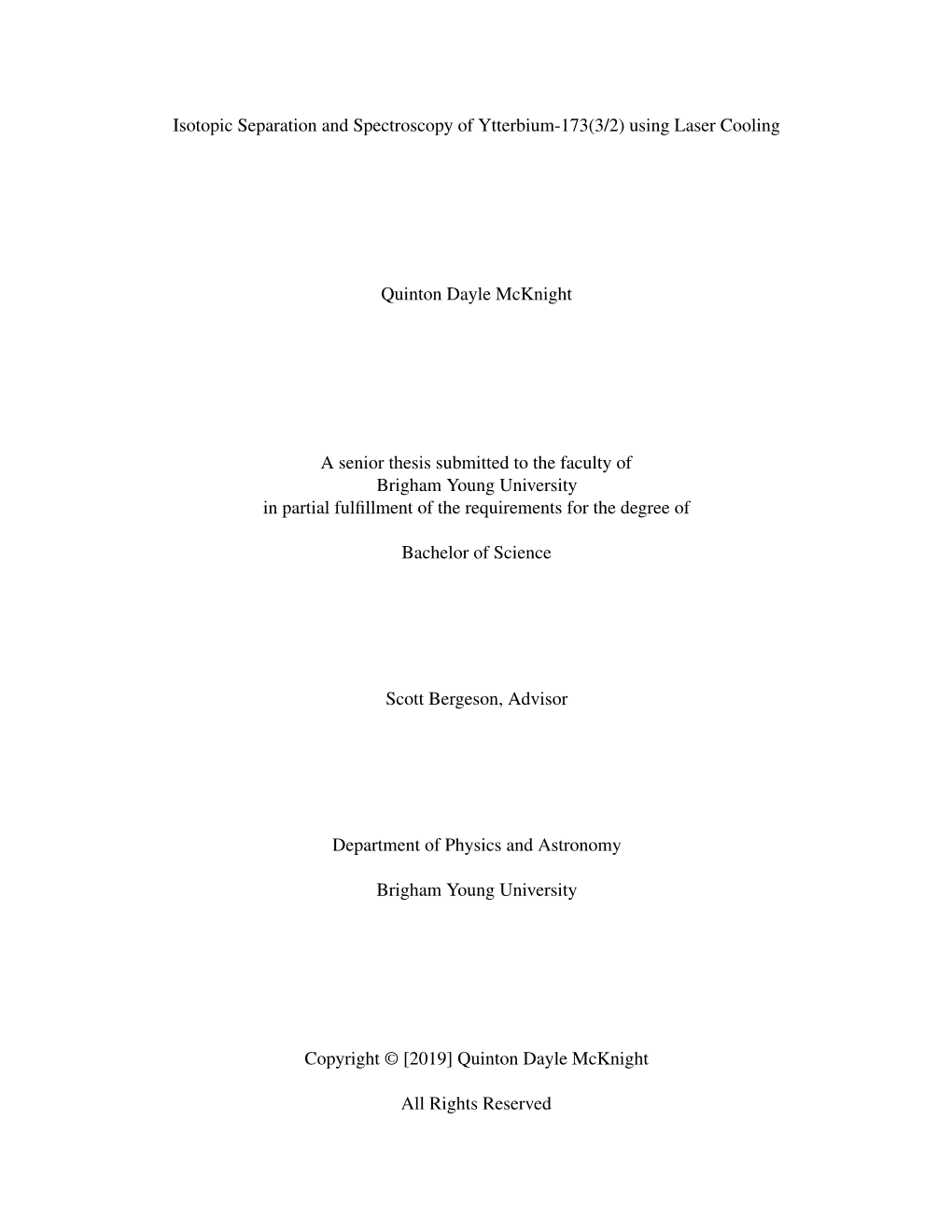
Load more
Recommended publications
-
Nuclear Energy Agency Nuclear Data Committee
NUCLEAR ENERGY AGENCY NUCLEAR DATA COMMITTEE SUMMARY RECORD OF THE lWEEFPY-FIRST MEETING (Technical Sessions) CBNM, Gee1 (Belgium) 24th-28th September 1979 Compiled by C. COCEVA (Scientific Secretary) OECD NUCLEAR ENERGY AGENCY 38 Bd. Suchet, 75016 Paris TABLE OF CONTENTS TECHNICAL SESSIONS Participants in meeting 1. Isotopes 2. National Progress Reports 3. Meetings 4. Technical Discussions 5. Topical Meeting on "Progress in Neutron Data of Structural Materials for Fast ~eactors" 6. Neutron and Related Nuclear Data Compilations and Evaluations Appendices 1 Meetings of the IAEA/NDS planned for 1980, 1981 and 1982 2 Progranme of the Topical Meeting on "Progress in Neutron Data of Structural Materials for Fast Reactors " 3 Summary of the general discussion on the works presented at the Topical Meeting TECmTICAL SESSIONS Perticipants in the 21st Meeting were as follows : For Canada : Dr. W.G. Cross Atomic Energy of Canada Ltd. Chalk River For Japan : Dr. K. Tsukada Japan Atomic Energy Research Institute Tokai-blur a For the United States of America : Dr. R.E. Chrien (Chairman) Brookhaven National Laboratory Dr. S.L. Wl~etstone U.S. Department of Energy Dr. 8.T. Motz Los Alamos Scientific Laboratory Dr. F.G. Perey Oak Ridge National Laboratory For the countries of the European Communities and the European Commission acting together : Dr. R. Iiockhoff (Local Secretary) Central Bureau for Nuclear Pleasurements Geel, Belgium Dr. C. Coceva (Scientific Secretary) Comitato Nazionale per 1'Energia Nucleare Bologna, Italy Dr. S. Cierjacks Kernforschungszentrum Karlsruhe Federal Republic of Germany Dr. C. Fort Conunissariat i 1'Energie Atomique Cadaroche, France Dr. A. Michaudon (Vice-chairman) Commissariat 2 1'Energie Atomique Bruysrcs-1.e-ChZtel Dr. -
Metastable Non-Nucleonic States of Nuclear Matter: Phenomenology
Physical Science International Journal 15(2): 1-25, 2017; Article no.PSIJ.34889 ISSN: 2348-0130 Metastable Non-Nucleonic States of Nuclear Matter: Phenomenology Timashev Serge 1,2* 1Karpov Institute of Physical Chemistry, Moscow, Russia. 2National Research Nuclear University MEPhI, Moscow, Russia. Author’s contribution The sole author designed, analyzed and interpreted and prepared the manuscript. Article Information DOI: 10.9734/PSIJ/2017/34889 Editor(s): (1) Prof. Yang-Hui He, Professor of Mathematics, City University London, UK And Chang-Jiang Chair Professor in Physics and Qian-Ren Scholar, Nan Kai University, China & Tutor and Quondam-Socius in Mathematics, Merton College, University of Oxford, UK. (2) Roberto Oscar Aquilano, School of Exact Science, National University of Rosario (UNR),Rosario, Physics Institute (IFIR)(CONICET-UNR), Argentina. Reviewers: (1) Alejandro Gutiérrez-Rodríguez, Universidad Autónoma de Zacatecas, Mexico. (2) Arun Goyal, Delhi University, India. (3) Stanislav Fisenko, Moscow State Linguistic University, Russia. Complete Peer review History: http://www.sciencedomain.org/review-history/20031 Received 17 th June 2017 Accepted 8th July 2017 Original Research Article th Published 13 July 2017 ABSTRACT A hypothesis of the existence of metastable states for nuclear matter with a locally shaken-up nucleonic structure of the nucleus, was proposed earlier. Such states are initiated by inelastic scattering of electrons by nuclei along the path of weak nuclear interaction. The relaxation of such nuclei is also determined by weak interactions. The use of the hypothesis makes it possible to physically interpret a rather large group of experimental data on the initiation of low energy nuclear reactions (LENRs) and the acceleration of radioactive α- and β-decays in a low-temperature plasma. -
CROSS SECTIONS of (N,P), (N,Α), (N,2N) REACTIONS on ISOTOPES
CROSS SECTIONS OF (n, p), (n, α), (n, 2n) REACTIONS ON ISOTOPES OF Dy, Er, Yb AT En= 14.6 MEV А.O. Kadenko, O.M. Gorbachenko, V.A. Plujko, G.I. Primenko Nuclear Physics Department, Taras Shevchenko National University, Kyiv, Ukraine Abstract The cross sections of the neutron reactions at En= 14.6 MeV on the isotopes of Dy, Er, Yb with emission of neutrons, proton and alpha-particle were studied by the use of new experimental data and different theoretical approaches. New and improved experimental data were obtained by the neutron- activation technique. Present experimental results and evaluated nuclear data from EXFOR, TENDL, ENDF data libraries were compared with different systematics and calculations within codes of EMPIRE 3.0 and TALYS 1.2. Contribution of pre-equilibrium decay was studied. The recommendations on validity of different systematics for estimations of cross-sections of considered reactions are given. 1. Introduction Studies of the nuclear reaction cross sections induced by neutrons provides an opportunity to get information on the excited states of atomic nuclei and nuclear reaction mechanisms [1]. In addition, nuclear reaction cross section data are necessary in applied applications such as the design of fusion reactors protection and modernization of existing nuclear power plants [2, 3]. In particular, they allow to calculate the activity and the degree of radiation damage to structural elements of nuclear reactors [3]. Practical interest in cross sections of nuclear reactions caused by their wide use in experimental nuclear physics methods, such as the method of boundary indicators in measuring the spectrum of neutrons in the reactor core of a nuclear reactor, or neutron activation analysis. -
Abstract Ultracold Mixtures of Rubidium and Ytterbium
ABSTRACT Title of dissertation: ULTRACOLD MIXTURES OF RUBIDIUM AND YTTERBIUM FOR OPEN QUANTUM SYSTEM ENGINEERING Creston David Herold, Doctor of Philosophy, 2014 Dissertation directed by: Dr. James Porto and Professor Steven Rolston Joint Quantum Institute, University of Maryland Department of Physics and National Institute of Standards and Technology Exquisite experimental control of quantum systems has led to sharp growth of basic quantum research in recent years. Controlling dissipation has been crucial in producing ultracold, trapped atomic samples. Recent theoretical work has suggested dissipation can be a useful tool for quantum state preparation. Controlling not only how a system interacts with a reservoir, but the ability to engineer the reservoir itself would be a powerful platform for open quantum system research. Toward this end, we have constructed an apparatus to study ultracold mixtures of rubidium (Rb) and ytterbium (Yb). We have developed a Rb-blind optical lattice at λzero = 423:018(7) nm, which will enable us to immerse a lattice of Yb atoms (the system) into a Rb BEC (superfluid reservoir). We have produced Bose-Einstein condensates of 170Yb and 174Yb, two of the five bosonic isotopes of Yb, which also has two fermionic isotopes. Flexible optical trapping of Rb and Yb was achieved with a two-color dipole trap of 532 and 1064 nm, and we observed thermalization in ultracold mixtures of Rb and Yb. Using the Rb-blind optical lattice, we measured very small light shifts of 87Rb BECs near the λzero wavelengths adjacent the 6p electronic states, through a coherent series of lattice pulses. The positions of the λzero wavelengths are sensitive to the electric dipole matrix elements between the 5s and 6p states, and we made the first experimental measurement of their strength. -
Detection of Elements at All Three R-Process Peaks in the Metal-Poor Star HD 160617
Published in the Astrophysical Journal A Preprint typeset using LTEX style emulateapj v. 5/2/11 DETECTION OF ELEMENTS AT ALL THREE R-PROCESS PEAKS IN THE METAL-POOR STAR HD 1606171 ,2 ,3 Ian U. Roederer4 and James E. Lawler5 Published in the Astrophysical Journal ABSTRACT We report the first detection of elements at all three r-process peaks in the metal-poor halo star HD 160617. These elements include arsenic and selenium, which have not been detected previously in halo stars, and the elements tellurium, osmium, iridium, and platinum, which have been detected previously. Absorption lines of these elements are found in archive observations made with the Space Telescope Imaging Spectrograph onboard the Hubble Space Telescope. We present up-to-date absolute atomic transition probabilities and complete line component patterns for these elements. Additional archival spectra of this star from several ground-based instruments allow us to derive abundances or upper limits of 45 elements in HD 160617, including 27 elements produced by neutron-capture reactions. The average abundances of the elements at the three r-process peaks are similar to the predicted solar system r-process residuals when scaled to the abundances in the rare earth element domain. This result for arsenic and selenium may be surprising in light of predictions that the production of the lightest r-process elements generally should be decoupled from the heavier r-process elements. Subject headings: atomic data — nuclear reactions, nucleosynthesis, abundances — stars: abundances — stars: individual (HD 160617) — stars: Population II 1. INTRODUCTION in greater abundance during n-capture reactions. The Understanding the origin of the elements is one of the s-process path closely follows the valley of β-stability, major challenges of modern astrophysics. -
Fi Oooc U 1999 3 1 / 40
fi OooC PL0002050 ISSN 1425-204X INSTITUTE OF NUCLEAR CHEMISTRY AND TECHNOLOGY SL ¥ u 1999 31/ 40 Please be aware that all of the Missing Pages in this document were originally blank pages EDITORS Wiktor Smuiek, Ph.D. Ewa Godlewska-Para PRINTING Sylwester Wojtas © Copyright by the Institute of Nuclear Chemistry and Technology, Warszawa 2000 All rights reserved CONTENTS GENERAL INFORMATION 9 MANAGEMENT OF THE INSTITUTE 11 MANAGING STAFF OF THE INSTITUTE 11 HEADS OF THE INCT DEPARTMENTS 11 SCIENTIFIC COUNCIL (1999-2003) 11 SCIENTIFIC STAFF 14 PROFESSORS 14 ASSOCIATE PROFESSORS 14 SENIOR SCIENTISTS (Ph.D.) 14 RADIATION CHEMISTRY AND PHYSICS, RADIATION TECHNOLOGIES 17 GENERATION OF RADICAL CATIONS FROM PHENYL, VINYL, AND ALLYL CONTAINING THIOETHERS IN ORGANIC SOLVENTS A. Korzeniowska-Sobczuk, P. Wiśniewski, K. Bobrowski, L. Richter, O. Brede 19 EPR STUDIES OF RADICALS INDUCED BY IONISING RADIATION IN FLUTAMIDE H.B. Ambroż, E. Kornacka, G. Przybytniak 20 THE ROLE OF Cu(I) AND Cu(II) IN DNA DAMAGES H.B. Ambroż, E. Kornacka, G. Przybytniak 21 TEMPERATURE COEFFICIENT OF THE RADIATION YIELD OF THE RADICAL CH3 • CH COf IN CRYSTALLINE ALANINĘ Z.P. Zagórski 22 COMPETITION BETWEEN INTRAMOLECULAR TWO-CENTERED THREE-ELECTRON BONDED (S. • .S)+ AND (S. • .N)+ FORMATION DURING PHOTOOXIDATION OF METHIONINE-CONTAINING PEPTIDES BY THE 4-CARBOXYBENZOPHENONE TRIPLET STATE IN AQUEOUS SOLUTION K. Bobrowski, G.L. Hug, H. Kozubek, B. Marciniak 23 Trp[NH ' +] -Tyr[O ' ] RADICAL TRANSFORMATION IN H-Trp-(Pro)n-Tyr-OH,n = 3-5, SERIES OF PEPTIDES K. Bobrowski, J. Poznański, J. Holcman, K.L. Wierzchowski 25 EPR OF METALS NANOPARTICLES IN MCM-41 MOLECULAR SIEVES J. -
Optical Frequency Measurement and Ground State Cooling of Single Trapped Yb+ Ions
Optical frequency measurement and ground state cooling of single trapped Yb+ ions Peter Blythe March 2004 with updates and corrections August 2005 Submitted in partial ful¯lment of the requirements for the degree of Doctor of Philosophy of the University of London Abstract The thesis describes experiments on single laser-cooled ions of Yb+ in a radiofrequency ion trap. 2 2 The ion is laser-cooled on the 369 nm S1=2! P1=2 electric dipole tran- 2 2 sition, and high resolution spectroscopy of the 467 nm S1=2! F7=2 electric octupole `clock' transition has been performed. The 467 nm transition can be used as an optical frequency standard. To this end, several absolute optical frequency measurements of the F = 171 + 0; mF = 0 ! F = 3; mF = 0 component in Yb have been made with a femtosecond laser frequency comb generator. The comb was referenced to a hydrogen maser which forms part of the clock ensemble used to generate the UTC(NPL) timescale. During the work described in this thesis, the linewidth of the 467 nm probe laser has been narrowed from 4 kHz to 200 Hz, increasing the measurement resolution. The frequency measurements have been supported by a full investigation of the systematic frequency shifts of the octupole transition, including the AC Stark, second-order Zeeman, quadrupole, DC Stark, second-order Doppler and blackbody shifts. Cooling an ion of 172Yb+ to the ground motional state of the ion trap by `EIT cooling' on the 369 nm transition has been investigated, both by numerical simulation and experiment. A new technique for measuring the temperature of a trapped ion without the use of a narrow transition has been developed. -
A Two-Orbital Quantum Gas with Tunable Interactions
A two-orbital quantum gas with tunable interactions Moritz Höfer München 2017 A two-orbital quantum gas with tunable interactions Dissertation an der Fakultät für Physik Ludwig-Maximilians-Universität München vorgelegt von Moritz Höfer aus Stade München, den 02. März 2017 Tag der mündlichen Prüfung: 7. April 2017 Erstgutachter: Prof. Immanuel Bloch Zweitgutachter: Prof. Wilhelm Zwerger Weitere Prüfungskommissionsmitglieder: Prof. A. Högele, Prof. M. Punk Zusammenfassung Im letzten Jahrzehnt haben sich Quantengasexperimente als gut kontrollierbare Modell- systeme zur Untersuchung komplexer Fragestellungen aus diversen Bereichen der Physik etabliert. Ultrakalte Quantengase zeichnen sich insbesondere dadurch aus, dass sie einen direkten und experimentell einfach realisierbaren Zugang zu ihrer Wechselwirkung bieten. Das gezielte Einstellen der Wechselwirkungsstärke und die Erforschung der daraus resul- tierenden Aggregatzustände erlaubt es ein tiefes Verständnis der kondensierten Materie zu gewinnen. Insbesondere erdalkaliähnliche Atome wie Ytterbium bieten die Möglich- keit Phänomene der Festkörperphysik zu untersuchen, die durch die Wechselwirkung von Elektronen in verschiedenen Orbitalen oder durch eine größere Rotationssymmetrie des Spins als in gewöhnlichen Spin-1/2 Systemen hervorgerufen werden. Diese Doktorarbeit präsentiert die experimentelle Charakterisierung der Wechselwir- kung ultrakalter, fermionischer Ytterbium-Atome (173Yb) in verschiedenen elektronischen Orbitalen. Dabei wird nachgewiesen, dass sich die Wechselwirkungsstärke -
Quantum Nanophotonics with Ytterbium in Yttrium Orthovanadate
Quantum nanophotonics with ytterbium in yttrium orthovanadate Thesis by Jonathan Miners Kindem In Partial Fulfillment of the Requirements for the Degree of Doctor of Philosophy CALIFORNIA INSTITUTE OF TECHNOLOGY Pasadena, California 2019 Defended March 14, 2019 ii © 2019 Jonathan Miners Kindem ORCID: 0000-0002-7737-9368 All rights reserved. iii ACKNOWLEDGEMENTS Thank you Andrei for being such a wonderful advisor throughout my time at Cal- tech. Your enthusiasm and excitement for doing science is contagious. Thanks for your patience as I climbed out of various “pits of incompetency" over the years and for having a good sense of humor. Thank you for setting high expectations for our work while giving me freedom in the lab to pursue things I found interesting. It’s been a lot of fun. Thank you to the Faraon group: Tian for getting me up to speed in the lab and teaching me so much over the years. Evan for all the late-night chats in the office and trips to the climbing gym. John for always being willing to answer “quick questions” that were never really quick questions and for your enthusiasm for rare- earths. Jake for keeping the rare-earth ego in check and for fabricating nanobeams. Andrei R. for bringing a fresh set of eyes to the experiment and for the fun times getting the singles measurements working. I’m glad to be leaving the experiment in good hands and look forward to seeing where experiments go in the future. Ioana for always being a source of positivity in lab and for letting me monopolize the fridge over the last few months. -
Production and Chemical Processing of 177Lu for Nuclear Medicine at the Munich Research Reactor FRM-II
Institut f¨urRadiochemie Production and chemical processing of 177Lu for nuclear medicine at the Munich research reactor FRM-II Dissertation of Zuzana Dvoˇr´akov´a Technische Universit¨atM¨unchen Institut f¨urRadiochemie der Technischen Universit¨atM¨unchen Production and chemical processing of 177Lu for nuclear medicine at the Munich research reactor FRM-II Zuzana Dvoˇr´akov´a Vollst¨andiger Abdruck der von der Fakult¨at f¨urChemie der Technischen Universit¨atM¨unchen zur Erlangung des akademischen Grades eines Doktors der Naturwissenschaften (Dr. rer. nat.) genehmigten Dissertation. Vorsitzender: Univ.-Prof. Dr. Dr. h. c. B. Rieger Pr¨ufer der Dissertation: 1. Univ.-Prof. Dr. A. T¨urler 2. Univ.-Prof. Dr. K. K¨ohler Die Dissertation wurde am 13.8.2007 bei der Technischen Universit¨at M¨unchen eingere- icht und durch die Fakult¨at f¨urChemie am 20.9.2007 angenommen. Abstract The main goal of the present dissertation was to investigate the feasibility of pro- ducing the radionuclide 177Lu at the Munich research reactor FRM-II. Lutetium-177 represents an ideal therapeutic radioisotope with suitable chemical properties and nuclear decay characteristics. Radiopharmaceuticals containing 177Lu are currently under development and tested for the treatment of various cancers in dozens of clin- ical trials around the world. The radionuclide 177Lu can be produced either directly by the 176Lu(n, γ)177Lu β− reaction or indirectly by the 176Yb(n, γ)177Yb → 177Lu reaction. The irradiation yield of 177Lu in both production routes was experimentally determined and compared with theoretical calculations. A reliable method for the calculation of the 177Lu yield, based on the Westcott convention, was established, which is more accurate than the methods published in the literature. -
T H Is D Is S E R Ta Tio N Has Been G1—5072 M Ic R O F Ilm E D E X Actly As
This dissertation has been G1—5072 microfilmed exactly as received BOWMAN, Jr., George Br'ice, 1932- DECON TAMIN ATION OF SOME SE EEC TED BABE EARTH ISOTOPES AND T1IE GAMMA RADIATIONS OF HAFNIUM-172 AND -173. The Ohio State University, Ph.D., 1961 Physics, nuclear University Microfilms, Inc., Ann Arbor, Michigan IECQNTAMINATICffi OF SOME SELECTED RARE EARTH ISOTOPES AMD THE GAMMA RADIATIONS OP HAIOTUM-172 AND -175 DISSERTATION Presented in Partial Fulfillment of the Requirements for the Degree Doctor of Philosophy in the Graduate School of The Ohio State University By George Price Bovaan, Jr., B. S., A. K. Capt&in, United States Air Force The Ohio State University 1961 Approved hy J / / A .......* / -it. i ..-'.v Adviser Department of Chemistry ■ ■ IV. .1 1 . ^ ^ .i.i A dviser Department of Physics ACXNCWIEDGMEHT The author wishes to express his appreciation to Professor M. H. Kurbatov and Professor J. D. Kurbatov for their guidance in this research, to Hr. H. J. Sathoff and Mr. P. J. Bradley for their assistance in collecting- the data, and to the United States Air Force for providing the opportunity for him to pursue this course of study. ii CONTENTS CHAPTER Page I CHEMICAL PURIFICATION OF SEIECTED RARE EARTH ISOTOPES . 1 Description of Cation-Exchange Columns ................................. 3 General Chemical Procedure for Decontamination of the Enriched Isotope Samples .................................................... 8 Chemical Procedure Flow Sheet .................................................... 11 P u rific a tio n of Neodymium-144 S a m p l e ................................. 12 P u rific a tio n of I>yspro3iui[h*156 S a m p l e ........................................14 Purification of Erbiunwl62 Sample ...... -
Atomic Parity Violation and Related Physics in Ytterbium by Dimitri
Atomic Parity Violation and Related Physics in Ytterbium by Dimitri Robert Dounas-Frazer A dissertation submitted in partial satisfaction of the requirements for the degree of Doctor of Philosophy in Physics in the Graduate Division of the University of California, Berkeley Committee in charge: Professor Dmitry Budker, Chair Professor Hartmut H¨affner Professor Robert Harris Fall 2012 Atomic Parity Violation and Related Physics in Ytterbium Copyright 2012 by Dimitri Robert Dounas-Frazer 1 Abstract Atomic Parity Violation and Related Physics in Ytterbium by Dimitri Robert Dounas-Frazer Doctor of Philosophy in Physics University of California, Berkeley Professor Dmitry Budker, Chair 2 1 3 Atomic parity violation has been observed in the 408 nm 6s S0 ! 5d6s D1 forbidden −10 transition of ytterbium. The parity-violating amplitude is 8:7(1:4) × 10 ea0, two orders of magnitude larger than in cesium, where the most precise experiments to date have been performed. This is in accordance with theoretical predictions and constitutes the largest atomic parity-violating amplitude yet observed. This also opens the way to future measure- ments of neutron skins and anapole moments by comparing parity-violating amplitudes for various isotopes and hyperfine components of the transition. We present a detailed description of the observation. Linearly polarized 408 nm light interacts with ytterbium atoms in crossed electric (E) and magnetic fields (B). The proba- 2 1 3 bility of the 6s S0 ! 5d6s D1 transition contains a parity-violating term, proportional to (E · B)[(E × E) · B], arising from interference between the amplitudes of transitions induced by the electroweak interaction and the Stark effect (E is the optical electric field).