Regulation of GABAAR Signaling and Neuroadaptations in Response to Diazepam by Joshua M. Lorenz-Guertin B.S. Grand Valley State
Total Page:16
File Type:pdf, Size:1020Kb
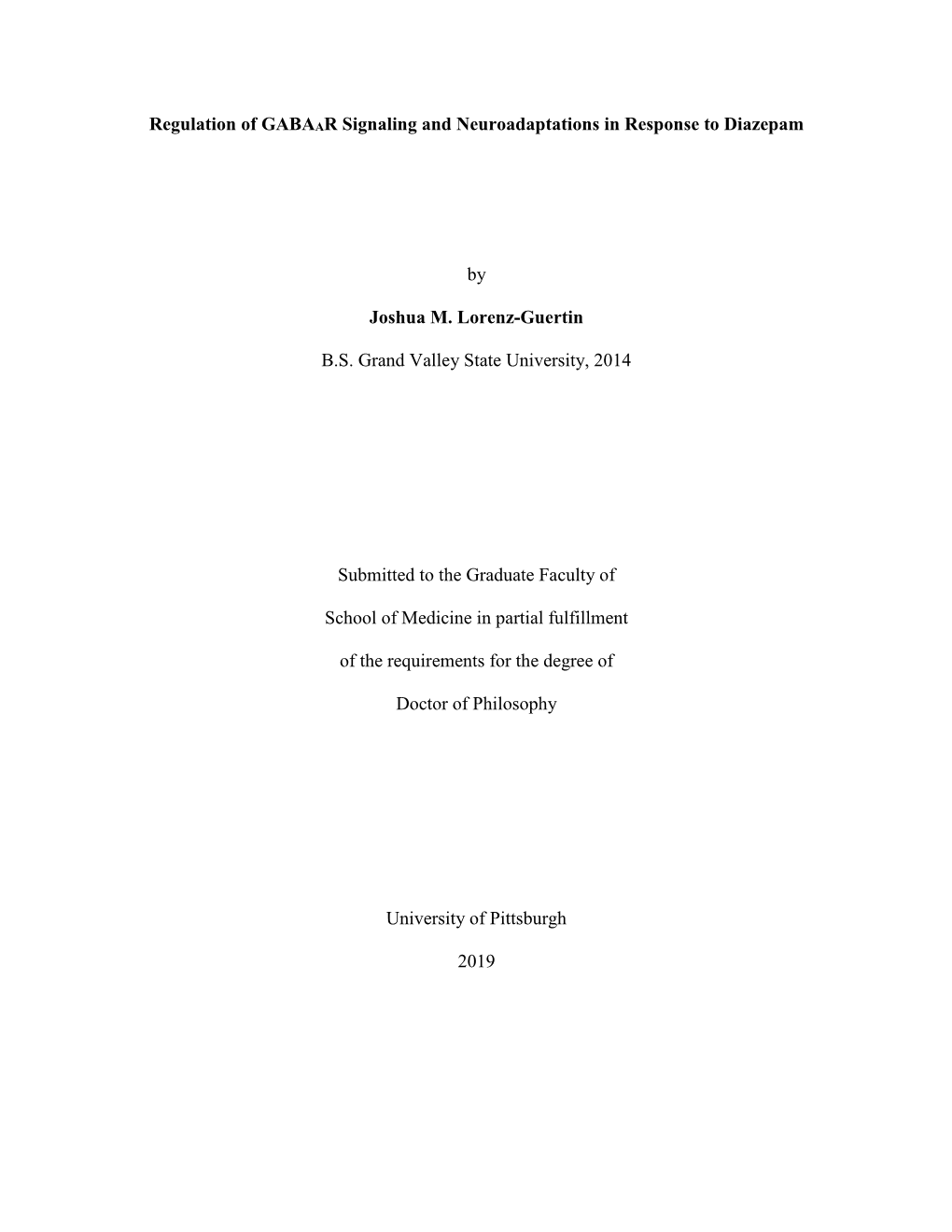
Load more
Recommended publications
-
A Large-Scale Conformational Change Couples Membrane Recruitment to Cargo Binding in the AP2 Clathrin Adaptor Complex
A Large-Scale Conformational Change Couples Membrane Recruitment to Cargo Binding in the AP2 Clathrin Adaptor Complex Lauren P. Jackson,1,5 Bernard T. Kelly,1,5 Airlie J. McCoy,1 Thomas Gaffry,2 Leo C. James,3 Brett M. Collins,4 Stefan Ho¨ ning,2 Philip R. Evans,3,* and David J. Owen1,* 1Cambridge Institute for Medical Research, Department of Clinical Biochemistry, University of Cambridge, Hills Road, Cambridge CB2 0XY, UK 2Institute of Biochemistry I and Center for Molecular Medicine Cologne, University of Cologne, Joseph-Stelzmann-Str. 52 50931 Cologne, Germany 3Medical Research Council Laboratory of Molecular Biology, Hills Road, Cambridge CB2 0QH, UK 4Institute for Molecular Bioscience, The University of Queensland, Brisbane QLD 4072, Australia 5These authors contributed equally to this work *Correspondence: [email protected] (P.R.E.), [email protected] (D.J.O.) DOI 10.1016/j.cell.2010.05.006 SUMMARY by clathrin adaptors to an outer polymeric clathrin scaffold (Cheng et al., 2007; Fotin et al., 2004). Clathrin adaptors contain The AP2 adaptor complex (a, b2, s2, and m2 sub- a folded membrane-proximal domain, which binds to phospha- units) crosslinks the endocytic clathrin scaffold to tidyl inositol polyphosphate (PIP) headgroups and/or Arf PtdIns4,5P2-containing membranes and transmem- GTPases in their membrane-attached, GTP-bound forms, and brane protein cargo. In the ‘‘locked’’ cytosolic form, at least one natively unstructured region, which harbors a cla- AP2’s binding sites for the two endocytic motifs, thrin-binding motif (Owen et al., 2004). Transmembrane proteins YxxF on the C-terminal domain of m2 (C-m2) and are generally selected as cargo for incorporation into a CCV through the direct interaction of either widely used, short, linear [ED]xxxL[LI] on s2, are blocked by parts of b2. -
Enzymatic Encoding Methods for Efficient Synthesis Of
(19) TZZ__T (11) EP 1 957 644 B1 (12) EUROPEAN PATENT SPECIFICATION (45) Date of publication and mention (51) Int Cl.: of the grant of the patent: C12N 15/10 (2006.01) C12Q 1/68 (2006.01) 01.12.2010 Bulletin 2010/48 C40B 40/06 (2006.01) C40B 50/06 (2006.01) (21) Application number: 06818144.5 (86) International application number: PCT/DK2006/000685 (22) Date of filing: 01.12.2006 (87) International publication number: WO 2007/062664 (07.06.2007 Gazette 2007/23) (54) ENZYMATIC ENCODING METHODS FOR EFFICIENT SYNTHESIS OF LARGE LIBRARIES ENZYMVERMITTELNDE KODIERUNGSMETHODEN FÜR EINE EFFIZIENTE SYNTHESE VON GROSSEN BIBLIOTHEKEN PROCEDES DE CODAGE ENZYMATIQUE DESTINES A LA SYNTHESE EFFICACE DE BIBLIOTHEQUES IMPORTANTES (84) Designated Contracting States: • GOLDBECH, Anne AT BE BG CH CY CZ DE DK EE ES FI FR GB GR DK-2200 Copenhagen N (DK) HU IE IS IT LI LT LU LV MC NL PL PT RO SE SI • DE LEON, Daen SK TR DK-2300 Copenhagen S (DK) Designated Extension States: • KALDOR, Ditte Kievsmose AL BA HR MK RS DK-2880 Bagsvaerd (DK) • SLØK, Frank Abilgaard (30) Priority: 01.12.2005 DK 200501704 DK-3450 Allerød (DK) 02.12.2005 US 741490 P • HUSEMOEN, Birgitte Nystrup DK-2500 Valby (DK) (43) Date of publication of application: • DOLBERG, Johannes 20.08.2008 Bulletin 2008/34 DK-1674 Copenhagen V (DK) • JENSEN, Kim Birkebæk (73) Proprietor: Nuevolution A/S DK-2610 Rødovre (DK) 2100 Copenhagen 0 (DK) • PETERSEN, Lene DK-2100 Copenhagen Ø (DK) (72) Inventors: • NØRREGAARD-MADSEN, Mads • FRANCH, Thomas DK-3460 Birkerød (DK) DK-3070 Snekkersten (DK) • GODSKESEN, -
Protein Sorting and Vesicular Traffic in the Golgi Apparatus
The Golgi Apparatus 63 E.G. Berger & J. Roth (eds) © 1997 Birkhauser Verlag Basel/Switzerland Protein sorting and vesicular traffic in the Golgi apparatus M.G. Farquhar1 and H.-P. Hauri2 JDivision ofCellular and Molecular Medicine and Department ofPathology, University ofCalifornia, San Diego, CA 92093-0651, USA 2Department ofPharmacology, Biozelltrum, University ofBasel, CH-4056 Basel, Switzerland Summary 65 Introduction 65 Major routes of protein and membrane traffic to and through the GA 66 Overview oftraffic along the exocytic pathway 66 Models ofGolgi organization 68 Mechanisms of sorting, targeting and transport from the ER 71 Exitfrom the ER occurs at specific export sites 71 Selective transportfrom the ER vs bulk-jlow models 72 Transit through the ER-Golgi intermediate compartment (ERGIC) 74 Other proximal pre-Golgi, pre-ERGIC, smooth ER compartments 76 Mechanisms for retention and retrieval ofresident ER proteins: Current models 79 Transport, processing and sorting by the GA 80 Contributions ofin vitro assays 82 The SNARE hypothesis 82 Golgi compartments and post-translational processing oftransported proteins 83 Golgi-specific functions . 83 How many Golgi compartments are there? 83 Proposed mechanisms for retention and retrieval ofresident Golgi proteins 86 Transmembrane domain retention signals 88 Formation ofinsoluble aggregates too large to enter transport vesicles 88 The kin recognition model 89 Bilayer-mediated sorting 89 Sorting at the TGN 90 Sorting oflysosomal enzymes 92 64 M.G. Farquhar and H.-P. Hauri Sorting and packaging -
Conserved and Novel Properties of Clathrin-Mediated Endocytosis in Dictyostelium Discoideum" (2012)
Rockefeller University Digital Commons @ RU Student Theses and Dissertations 2012 Conserved and Novel Properties of Clathrin- Mediated Endocytosis in Dictyostelium Discoideum Laura Macro Follow this and additional works at: http://digitalcommons.rockefeller.edu/ student_theses_and_dissertations Part of the Life Sciences Commons Recommended Citation Macro, Laura, "Conserved and Novel Properties of Clathrin-Mediated Endocytosis in Dictyostelium Discoideum" (2012). Student Theses and Dissertations. Paper 163. This Thesis is brought to you for free and open access by Digital Commons @ RU. It has been accepted for inclusion in Student Theses and Dissertations by an authorized administrator of Digital Commons @ RU. For more information, please contact [email protected]. CONSERVED AND NOVEL PROPERTIES OF CLATHRIN- MEDIATED ENDOCYTOSIS IN DICTYOSTELIUM DISCOIDEUM A Thesis Presented to the Faculty of The Rockefeller University in Partial Fulfillment of the Requirements for the degree of Doctor of Philosophy by Laura Macro June 2012 © Copyright by Laura Macro 2012 CONSERVED AND NOVEL PROPERTIES OF CLATHRIN- MEDIATED ENDOCYTOSIS IN DICTYOSTELIUM DISCOIDEUM Laura Macro, Ph.D. The Rockefeller University 2012 The protein clathrin mediates one of the major pathways of endocytosis from the extracellular milieu and plasma membrane. Clathrin functions with a network of interacting accessory proteins, one of which is the adaptor complex AP-2, to co-ordinate vesicle formation. Disruption of genes involved in clathrin-mediated endocytosis causes embryonic lethality in multicellular animals suggesting that clathrin-mediated endocytosis is a fundamental cellular process. However, loss of clathrin-mediated endocytosis genes in single cell eukaryotes, such as S.cerevisiae (yeast), does not cause lethality, suggesting that clathrin may convey specific advantages for multicellularity. -
Mechanisms of Synaptic Plasticity Mediated by Clathrin Adaptor-Protein Complexes 1 and 2 in Mice
Mechanisms of synaptic plasticity mediated by Clathrin Adaptor-protein complexes 1 and 2 in mice Dissertation for the award of the degree “Doctor rerum naturalium” at the Georg-August-University Göttingen within the doctoral program “Molecular Biology of Cells” of the Georg-August University School of Science (GAUSS) Submitted by Ratnakar Mishra Born in Birpur, Bihar, India Göttingen, Germany 2019 1 Members of the Thesis Committee Prof. Dr. Peter Schu Institute for Cellular Biochemistry, (Supervisor and first referee) University Medical Center Göttingen, Germany Dr. Hans Dieter Schmitt Neurobiology, Max Planck Institute (Second referee) for Biophysical Chemistry, Göttingen, Germany Prof. Dr. med. Thomas A. Bayer Division of Molecular Psychiatry, University Medical Center, Göttingen, Germany Additional Members of the Examination Board Prof. Dr. Silvio O. Rizzoli Department of Neuro-and Sensory Physiology, University Medical Center Göttingen, Germany Dr. Roland Dosch Institute of Developmental Biochemistry, University Medical Center Göttingen, Germany Prof. Dr. med. Martin Oppermann Institute of Cellular and Molecular Immunology, University Medical Center, Göttingen, Germany Date of oral examination: 14th may 2019 2 Table of Contents List of abbreviations ................................................................................. 5 Abstract ................................................................................................... 7 Chapter 1: Introduction ............................................................................ -
Gephyrin: a Scaffold That Builds a Phase at the Inhibitory Postsynapses
www.nature.com/cr www.cell-research.com RESEARCH HIGHLIGHT OPEN Gephyrin: a scaffold that builds a phase at the inhibitory postsynapses Christian Hoffmann 1 and Dragomir Milovanovic 1 Cell Research (2021) 31:245–246; https://doi.org/10.1038/s41422-020-00440-2 The scaffolding protein gephyrin is a core component of many GPHN-E. Besides GlyR-β, TM3–4 loops of GABAAR α3-subunit also inhibitory synapses. Bai and colleagues now show that phase phase separates with GPHN-E suggesting liquid condensation to separation of gephyrin with the subunits of both glycine and be a general feature of inhibitory synapses (Fig. 1). The phase GABA receptors underlies the formation of postsynaptic separation occurs at the equimolar ratio of receptors and scaffold sheets at the inhibitory synapses. proteins, a range similar to their physiological concentrations.8 To assure precise processing of information, contacts between There are several implications of this study. First, the observa- neurons require the alignment of the release sites, presynaptic tion that different regions of the same proteins (GlyR-β and GPHN- terminals, with the ionotropic transmitter receptors at the E) are important for phase separation or direct binding, suggests postsynaptic plasma membrane. Downstream of these receptors, that these two processes could be regulated separately. The scaffold molecules, kinases and cytoskeleton components all positively charged region of GlyR-β (a.a. 354–383) and negatively orchestrate the signaling cascade induced by the neurotransmitter charged surface at the subdomain II of GPHN-E are essential for fl 1234567890();,: binding and ion in ux. Each neuron in the mammalian central condensate formation, despite hydrophobic interactions being nervous system relies on both the excitatory and inhibitory inputs. -
And HPV18-Infected Early Stage Cervical Cancers and Normal
View metadata, citation and similar papers at core.ac.uk brought to you by CORE provided by Elsevier - Publisher Connector Virology 331 (2005) 269–291 www.elsevier.com/locate/yviro Gene expression profiles of primary HPV16- and HPV18-infected early stage cervical cancers and normal cervical epithelium: identification of novel candidate molecular markers for cervical cancer diagnosis and therapy Alessandro D. Santina,*, Fenghuang Zhanb, Eliana Bignottia, Eric R. Siegelc, Stefania Cane´ a, Stefania Bellonea, Michela Palmieria, Simone Anfossia, Maria Thomasd, Alexander Burnetta, Helen H. Kaye, Juan J. Romana, Timothy J. O’Briena, Erming Tianb, Martin J. Cannonf, John Shaughnessy Jr.b, Sergio Pecorellig aDivision of Gynecologic Oncology, University of Arkansas for Medical Sciences, Little Rock, AR 72205, USA bMyeloma Institute for Research and Therapy, University of Arkansas for Medical Sciences, Little Rock, AR 72205, USA cDepartment of Biostatistics, University of Arkansas for Medical Sciences, Little Rock, AR 72205, USA dDepartment of Pathology, University of Arkansas for Medical Sciences, Little Rock, AR 72205, USA eDepartment of Obstetrics and Gynecology, University of Arkansas for Medical Sciences, Little Rock, AR 72205, USA fDepartment of Microbiology and Immunology, University of Arkansas, Little Rock, AR 72205, USA gDivision of Gynecologic Oncology, University of Brescia, Brescia, Italy Received 2 July 2004; returned to author for revision 18 August 2004; accepted 9 September 2004 Available online 21 November 2004 Abstract With the goal of identifying genes with a differential pattern of expression between invasive cervical carcinomas (CVX) and normal cervical keratinocytes (NCK), we used oligonucleotide microarrays to interrogate the expression of 14,500 known genes in 11 primary HPV16 and HPV18-infected stage IB–IIA cervical cancers and four primary normal cervical keratinocyte cultures. -
Supplementary Table S4. FGA Co-Expressed Gene List in LUAD
Supplementary Table S4. FGA co-expressed gene list in LUAD tumors Symbol R Locus Description FGG 0.919 4q28 fibrinogen gamma chain FGL1 0.635 8p22 fibrinogen-like 1 SLC7A2 0.536 8p22 solute carrier family 7 (cationic amino acid transporter, y+ system), member 2 DUSP4 0.521 8p12-p11 dual specificity phosphatase 4 HAL 0.51 12q22-q24.1histidine ammonia-lyase PDE4D 0.499 5q12 phosphodiesterase 4D, cAMP-specific FURIN 0.497 15q26.1 furin (paired basic amino acid cleaving enzyme) CPS1 0.49 2q35 carbamoyl-phosphate synthase 1, mitochondrial TESC 0.478 12q24.22 tescalcin INHA 0.465 2q35 inhibin, alpha S100P 0.461 4p16 S100 calcium binding protein P VPS37A 0.447 8p22 vacuolar protein sorting 37 homolog A (S. cerevisiae) SLC16A14 0.447 2q36.3 solute carrier family 16, member 14 PPARGC1A 0.443 4p15.1 peroxisome proliferator-activated receptor gamma, coactivator 1 alpha SIK1 0.435 21q22.3 salt-inducible kinase 1 IRS2 0.434 13q34 insulin receptor substrate 2 RND1 0.433 12q12 Rho family GTPase 1 HGD 0.433 3q13.33 homogentisate 1,2-dioxygenase PTP4A1 0.432 6q12 protein tyrosine phosphatase type IVA, member 1 C8orf4 0.428 8p11.2 chromosome 8 open reading frame 4 DDC 0.427 7p12.2 dopa decarboxylase (aromatic L-amino acid decarboxylase) TACC2 0.427 10q26 transforming, acidic coiled-coil containing protein 2 MUC13 0.422 3q21.2 mucin 13, cell surface associated C5 0.412 9q33-q34 complement component 5 NR4A2 0.412 2q22-q23 nuclear receptor subfamily 4, group A, member 2 EYS 0.411 6q12 eyes shut homolog (Drosophila) GPX2 0.406 14q24.1 glutathione peroxidase -
Functional Characterization of Epilepsy Associated GABRG2 Mutations
Functional Characterization of Epilepsy Associated GABRG2 Mutations By Mengnan Tian Dissertation Submitted to the Faculty of the Graduate School of Vanderbilt University in Partial Fulfillment of the requirements for the degree of DOCTOR OF PHILOSOPHY in Pharmacology August, 2012 Nashville, Tennessee Approved: Robert L. Macdonald, M.D., Ph.D. Ronald Emeson, Ph.D. Kevin Ess, M.D., Ph.D. Katherine T. Murray, M.D. Douglas P. Mortlock, Ph.D. To my grandparents, Yunbo Tian and Zhenhua Cao. ii Acknowledgements Throughout my years pursuing Ph.D. degree, I have always been grateful for the opportunity working with an amazing complement of scientists. I would like to express my sincerest gratitude to my thesis advisor Dr. Robert Macdonald. He is by far the best mentor that I could have ever wished. He has provided me with excellent guidance and support, and given me the confidence to develop into an independent scientist. He granted me unprecedented freedom to explore new scientific fields and implement novel research strategies and techniques. I greatly appreciate all his trust in me, and it has fostered my confidence in performing researches. He has shown me, by his example, what a good scientist and mentor should be. I would like to thank my colleagues and collaborators in Macdonald lab. I have been collaborating with Xuan Huang since her rotation and after she joined our lab in 2009. We have also become good friends. She has made important contributions to many parts of my thesis studies, and provided brilliant critiques to help me improve the research plan. I am also deeply indebted to Dr. -
Supplemental Table S1
Electronic Supplementary Material (ESI) for Molecular Omics. This journal is © The Royal Society of Chemistry 2020 Supplemental table S1: List of proteins identified following MS analysis of the proteins removed of N-linked glycans and isolated from gels with an increased abundance in TIS Cal51 cells induced with paclitaxel versus control Cal51 cells. Protein in increased abundance in TIS vs control WCL Accession Fold Change Anova (P) Plectin Q15149 1.073855593 0.00691631 Ras GTPase-activating-like protein IQGAP1 P46940 1.087337643 0.0176342 Elongation factor1-gamma P26641 1.138709703 0.0116496 Peptidyl-prolyl cis-transisomerase B P23284 1.188383105 0.0436246 Dipeptidyl peptidase 3 Q9NY33 1.20163605 0.0215448 Transitional endoplasmic reticulum ATPase P55072 1.214194884 0.0449691 Carbonic anhydrase 2 P00918 1.232852325 0.0158141 Clathrin heavy chain 1 Q00610 1.239621773 0.0463237 Protein transport protein Sec 31A O94979 1.263565104 0.0284155 Aldo-ketoreductase family 1 member C1 Q04828 1.282092186 0.0324406 Spermidine synthase P19623 1.298728621 0.0196232 Plastin-3 P13797 1.310756772 0.0161319 Actin-related protein 2/3 complex subunit 5 O15511 1.333483524 0.00476923 Actin-related protein 2/3 complex subunit 2 O15144 1.35416168 0.0411018 Proteasome subunit alpha type-5 P28066 1.358015551 0.0337657 Thioredoxin reductase 1, cytoplasmic Q16881 1.383670089 0.0235472 Acyl-protein thioesterase 2 O95372 1.387415589 0.00233899 Isoaspartylpeptidase/L-asparaginase Q7L266 1.408149002 0.0319602 Splicing factor U2AF 65kDa subunit P26368 1.41489991 0.0256619 -
RNA-Seq Transcriptome Reveals Different Molecular Responses
Zhao et al. BMC Genomics (2020) 21:475 https://doi.org/10.1186/s12864-020-06885-4 RESEARCH ARTICLE Open Access RNA-Seq transcriptome reveals different molecular responses during human and mouse oocyte maturation and fertilization Zheng-Hui Zhao1,2, Tie-Gang Meng1,3, Ang Li1, Heide Schatten4, Zhen-Bo Wang1,2* and Qing-Yuan Sun1,3* Abstract Background: Female infertility is a worldwide concern and the etiology of infertility has not been thoroughly demonstrated. Although the mouse is a good model system to perform functional studies, the differences between mouse and human also need to be considered. The objective of this study is to elucidate the different molecular mechanisms underlying oocyte maturation and fertilization between human and mouse. Results: A comparative transcriptome analysis was performed to identify the differentially expressed genes and associated biological processes between human and mouse oocytes. In total, 8513 common genes, as well as 15, 165 and 6126 uniquely expressed genes were detected in human and mouse MII oocytes, respectively. Additionally, the ratios of non-homologous genes in human and mouse MII oocytes were 37 and 8%, respectively. Functional categorization analysis of the human MII non-homologous genes revealed that cAMP-mediated signaling, sister chromatid cohesin, and cell recognition were the major enriched biological processes. Interestingly, we couldn’t detect any GO categories in mouse non-homologous genes. Conclusions: This study demonstrates that human and mouse oocytes exhibit significant differences in gene expression profiles during oocyte maturation, which probably deciphers the differential molecular responses to oocyte maturation and fertilization. The significant differences between human and mouse oocytes limit the generalizations from mouse to human oocyte maturation. -
Clathrin-Mediated Endocytosis at Synapses
# 2007 The Authors Journal compilation # 2007 Blackwell Publishing Ltd Traffic 2007; 8: 1129–1136 Blackwell Munksgaard doi: 10.1111/j.1600-0854.2007.00595.x Review Clathrin-Mediated Endocytosis at Synapses Nadja Jung and Volker Haucke* We will describe first the role of CME in recycling of presynaptic vesicles and in regulating the number of Department of Membrane Biochemistry, Institute of surface-active neurotransmitter (NT) receptors. Then, we Chemistry & Biochemistry, Freie Universita¨t Berlin, will elaborate on the molecular mechanisms by which Takustraße 6, 14195 Berlin, Germany clathrin-associated adaptor proteins recognize and inter- *Corresponding author: Volker Haucke, nalize synaptic cargo destined for endocytosis. Based on [email protected] these insights, an arsenal of molecular weapons has been used to monitor clathrin-dependent vesicle cycling at Neurons are communication specialists that convert synapses or to arrest CME at morphologically and physio- electrical into chemical signals at specialized cell–cell logically discernible stages. junctions termed synapses. Arrival of an action potential triggers calcium-regulated exocytosis of neurotransmit- ter (NT) from small synaptic vesicles (SVs), which then diffuses across the synaptic cleft and binds to postsyn- Pathways of Synaptic Vesicle Recycling: aptic receptors to elicit specific changes within the post- The Tortoise and the Hare? synaptic cell. Endocytosis of pre- and postsynaptic membrane proteins including SV components and post- To sustain high-frequency NT release and to prevent synaptic NT receptors is essential for the proper func- expansion of the presynaptic plasmalemma, SVs must tioning of the synapse. During the past several years, we undergo local endocytic recycling within the nerve termi- have witnessed enormous progress in our understanding nal.