Dissecting the Contribution of Potassium Currents to the Heterogeneity in the Excitability Properties of Midbrain Dopamine Neuron Subpopulations
Total Page:16
File Type:pdf, Size:1020Kb
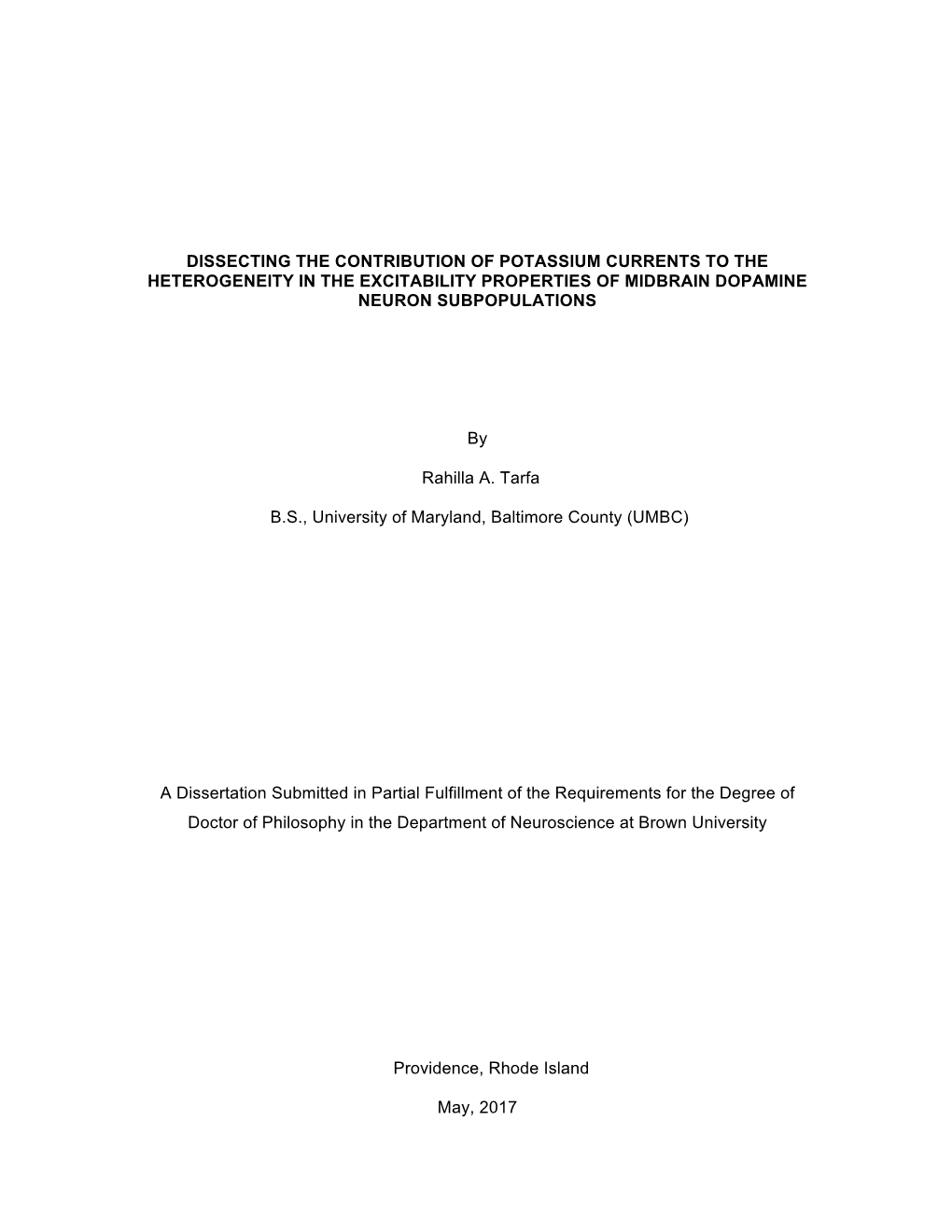
Load more
Recommended publications
-
Androctonus Mauretanicus Mauretanicus
Hindawi Publishing Corporation Journal of Toxicology Volume 2012, Article ID 103608, 9 pages doi:10.1155/2012/103608 Review Article Potassium Channels Blockers from the Venom of Androctonus mauretanicus mauretanicus Marie-France Martin-Eauclaire and Pierre E. Bougis Aix-Marseille University, CNRS, UMR 7286, CRN2M, Facult´edeM´edecine secteur Nord, CS80011, Boulevard Pierre Dramard, 13344 Marseille Cedex 15, France Correspondence should be addressed to Marie-France Martin-Eauclaire, [email protected] and Pierre E. Bougis, [email protected] Received 2 February 2012; Accepted 16 March 2012 Academic Editor: Maria Elena de Lima Copyright © 2012 M.-F. Martin-Eauclaire and P. E. Bougis. This is an open access article distributed under the Creative Commons Attribution License, which permits unrestricted use, distribution, and reproduction in any medium, provided the original work is properly cited. K+ channels selectively transport K+ ions across cell membranes and play a key role in regulating the physiology of excitable and nonexcitable cells. Their activation allows the cell to repolarize after action potential firing and reduces excitability, whereas channel inhibition increases excitability. In eukaryotes, the pharmacology and pore topology of several structural classes of K+ channels have been well characterized in the past two decades. This information has come about through the extensive use of scorpion toxins. We have participated in the isolation and in the characterization of several structurally distinct families of scorpion toxin peptides exhibiting different K+ channel blocking functions. In particular, the venom from the Moroccan scorpion Androctonus ffi + + mauretanicus mauretanicus provided several high-a nity blockers selective for diverse K channels (SKCa,Kv4.x, and Kv1.x K channel families). -
Stochastic and Deterministic Dynamics of Intrinsically Irregular Firing 3 in Cortical Inhibitory Interneurons
1 2 Stochastic and deterministic dynamics of intrinsically irregular firing 3 in cortical inhibitory interneurons 4 5 Philipe R.F. Mendonça1, Mariana Vargas-Caballero3, Ferenc Erdélyi2, Gábor Szabó2, 6 Ole Paulsen1 and Hugh P.C. Robinson1, 7 1Dept. of Physiology, Development and Neuroscience, University of Cambridge 8 2Division of Medical Gene Technology, Institute of Experimental Medicine, Budapest 9 3Institute for Life Sciences and Centre for Biological Sciences, University of 10 Southampton 11 12 Summary 13 Most cortical neurons fire regularly when excited by a constant stimulus. In contrast, 14 irregular-spiking (IS) interneurons are remarkable for the intrinsic variability of their spike 15 timing, which can synchronize amongst IS cells via specific gap junctions. Here, we have 16 studied the biophysical mechanisms of this irregular spiking in mice, and how IS cells fire in 17 the context of synchronous network oscillations. Using patch-clamp recordings, artificial 18 dynamic conductance injection, pharmacological analysis and computational modelling, we 19 show that spike time irregularity is generated by a nonlinear dynamical interaction of voltage- 20 dependent sodium and fast-inactivating potassium channels just below spike threshold, 21 amplifying channel noise. This active irregularity may help IS cells synchronize with each 22 other at gamma range frequencies, while resisting synchronization to lower input frequencies. 23 24 Running Title: Stochastic and deterministic mechanism of irregular spiking 25 1 26 HIGHLIGHTS 27 28 -
Refining the Identity and Role of Kv4 Channels in Mouse Substantia Nigra Dopaminergic Neurons
Research Article: Confirmation | Neuronal Excitability Refining the Identity and Role of Kv4 Channels in Mouse Substantia Nigra Dopaminergic Neurons https://doi.org/10.1523/ENEURO.0207-21.2021 Cite as: eNeuro 2021; 10.1523/ENEURO.0207-21.2021 Received: 10 May 2021 Accepted: 17 May 2021 This Early Release article has been peer-reviewed and accepted, but has not been through the composition and copyediting processes. The final version may differ slightly in style or formatting and will contain links to any extended data. Alerts: Sign up at www.eneuro.org/alerts to receive customized email alerts when the fully formatted version of this article is published. Copyright © 2021 Haddjeri-Hopkins et al. This is an open-access article distributed under the terms of the Creative Commons Attribution 4.0 International license, which permits unrestricted use, distribution and reproduction in any medium provided that the original work is properly attributed. ͳ REFINING THE IDENTITY AND ROLE OF KV4 CHANNELS IN MOUSE ʹ SUBSTANTIA NIGRA DOPAMINERGIC NEURONS ͵ Abbreviated Title: Kv4 channels in substantia nigra dopaminergic neurons Ͷ Alexis HADDJERI-HOPKINS1,*, Mónica TAPIA1,*, Jorge RAMIREZ-FRANCO1, Fabien TELL1,2, ͷ Béatrice MARQUEZE-POUEY1, Marianne AMALRIC3 and Jean-Marc GOAILLARD1,2,§ 1 UMR_S 1072, Aix Marseille University, INSERM, Faculté de Médecine Secteur Nord, 51 boulevard ͺ Pierre Dramard, 13015 Marseille, FRANCE ͻ 2 Current address: Institut de Neurosciences de la Timone, UMR 7289, Aix-Marseille Université and ͳͲ Centre National de la Recherche Scientifique, Marseille, FRANCE. ͳͳ 3 UMR7291, Aix Marseille University, CNRS, LNC, 3 place Victor Hugo, 13331 Marseille, FRANCE ͳʹ * These authors contributed equally to this work ͳ͵ § Corresponding author ͳͶ Corresponding author: Jean-Marc GOAILLARD. -
Ion Channels Product Listing | Edition 1
Ion Channels Product Listing | Edition 1 Western Honey Bee Apis mellifera A source of Apamin Products by Gating Mechanism: • Ligand-gated Ion Channels • Voltage-gated Ion Channels • Other Ion Channels Tocris Product Listing Series Introduction Ion channels are pore-forming proteins present in the plasma membrane of most cells, as well as the intracellular membranes surrounding many organelles, allowing the passage of ions across the membrane. They are essential for maintaining resting membrane potential, generating action potentials and other electrical signaling, and regulating cell volume. Ion channels have a fundamental role in physiological processes such as T cell activation, muscle contraction, insulin release from pancreatic β cells and transport of nutrients and ions across epithelial cells. A huge variety of ion channels exist, exhibiting selective permeability to particular ions and having different gating mechanisms. Ion channels may be classified according to their ion selectivity or gating mechanism, i.e. how the flow of ions is controlled. We have followed the IUPHAR classification of ion channels for the purposes of this listing and grouped the products into three main sections according to gating mechanism: Ligand-gated Ion Channels, Voltage- gated Ion Channels, and Other Channels. Contents Ligand-gated Ion Channels 3 Potassium Channels 17 Ca2+-Activated Potassium Channels 17 5-HT3 4 Inward Rectifier Potassium (K ) Channels 18 Acetylcholine Nicotinic Receptors 4 ir Two-P Potassium Channels 19 Nicotinic (α7) Receptors 4 -
Differential Robustness to Specific Potassium Channel Deletions In
bioRxiv preprint doi: https://doi.org/10.1101/845859; this version posted November 19, 2019. The copyright holder for this preprint (which was not certified by peer review) is the author/funder, who has granted bioRxiv a license to display the preprint in perpetuity. It is made available under aCC-BY 4.0 International license. DIFFERENTIAL ROBUSTNESS TO SPECIFIC POTASSIUM CHANNEL DELETIONS IN MIDBRAIN DOPAMINERGIC NEURONS Alexis HADDJERI-HOPKINS1, Béatrice MARQUEZE-POUEY1, Monica TAPIA1, Fabien TELL1, Marianne AMALRIC2 and Jean-Marc GOAILLARD1, 3 1 UMR_S 1072, Aix Marseille Université, INSERM, Faculté de Médecine Secteur Nord, Marseille, FRANCE 2 Aix Marseille Université, CNRS, LNC, FR3C, Marseille, FRANCEdetails 3 for Corresponding author DOI Author contributions manuscript: M.A. and J-M.G. designed research. A.H-H., B.M-P. and WITHDRAWNsee M.T. performed research. A.H-H., B.M-P., M.T. and F.T. analyzed data. A.H-H., F.T., M.A. and J-M.G. wrote the manuscript. Corresponding author: Jean-Marc GOAILLARD. UMR_S 1072, Aix Marseille Université, INSERM, Faculté de Médecine Secteur Nord, Marseille, FRANCE. Email: [email protected] Conflict of interest The authors declare no competing interests. 1 bioRxiv preprint doi: https://doi.org/10.1101/845859; this version posted November 19, 2019. The copyright holder for this preprint (which was not certified by peer review) is the author/funder, who has granted bioRxiv a license to display the preprint in perpetuity. It is made available under aCC-BY 4.0 International license. Abstract Quantifying the level of robustness of neurons in ion channel knock-out (KO) mice depends on how exhaustively electrical phenotype is assessed. -
Differential Robustness to Specific Potassium Channel
DIFFERENTIAL ROBUSTNESS TO SPECIFIC POTASSIUM CHANNEL DELETIONS IN MIDBRAIN DOPAMINERGIC NEURONS Alexis Haddjeri-Hopkins, Béatrice Marqueze-Pouey, Monica Tapia, Fabien Tell, Marianne Amalric, Jean-Marc Goaillard To cite this version: Alexis Haddjeri-Hopkins, Béatrice Marqueze-Pouey, Monica Tapia, Fabien Tell, Marianne Amalric, et al.. DIFFERENTIAL ROBUSTNESS TO SPECIFIC POTASSIUM CHANNEL DELETIONS IN MIDBRAIN DOPAMINERGIC NEURONS. 2020. hal-03026595 HAL Id: hal-03026595 https://hal.archives-ouvertes.fr/hal-03026595 Preprint submitted on 26 Nov 2020 HAL is a multi-disciplinary open access L’archive ouverte pluridisciplinaire HAL, est archive for the deposit and dissemination of sci- destinée au dépôt et à la diffusion de documents entific research documents, whether they are pub- scientifiques de niveau recherche, publiés ou non, lished or not. The documents may come from émanant des établissements d’enseignement et de teaching and research institutions in France or recherche français ou étrangers, des laboratoires abroad, or from public or private research centers. publics ou privés. DIFFERENTIAL ROBUSTNESS TO SPECIFIC POTASSIUM CHANNEL DELETIONS IN MIDBRAIN DOPAMINERGIC NEURONS Alexis HADDJERI-HOPKINS1, Béatrice MARQUEZE-POUEY1, Monica TAPIA1, Fabien TELL1, Marianne AMALRIC2 and Jean-Marc GOAILLARD1, 3 1 UMR_S 1072, Aix Marseille Université, INSERM, Faculté de Médecine Secteur Nord, Marseille, FRANCE 2 Aix Marseille Université, CNRS, LNC, FR3C, Marseille, FRANCE 3 Corresponding author Author contributions : M.A. and J-M.G. designed research. A.H-H., B.M-P. and M.T. performed research. A.H-H., B.M-P., M.T. and F.T. analyzed data. A.H-H., F.T., M.A. and J-M.G. wrote the manuscript. -
Doctoral Dissertation
UNIVERSITY OF COPENH AGEN FACULTY OF HEALTH A ND MEDICAL SCIENCES Doctoral Dissertation Kirstine Callø The Transient Outward Potassium Current in Healthy and Diseased Hearts December 2017 The Faculty of Health and Medical Sciences at the University of Copenhagen has accepted this dissertation, which consists of the already published dissertations listed below, for public defence for the doctoral degree in Veterinary Science. Copenhagen, 24th of October 2018. Professor Ulla Wewer, Dean Public defence will be held in auditorium A1-05.01, Dyrlægevej 100, Frederiksberg Campus, University of Copenhagen, Friday 11th of January 2019 at 1 p.m. Kirstine Callø Section for Anatomy, Biochemistry and Physiology Department of Veterinary and Animals Sciences Faculty of Health and Medical Sciences University of Copenhagen [email protected] Copenhagen 30.12.2018 ISBN 978-87-971049-0-3 1 1 Contents 2 PUBLICATIONS..................................................................................................................... 3 3 PREFACE .............................................................................................................................. 4 3.1 Conflict of interests ........................................................................................................................................ 4 4 DANSK RESUMÉ................................................................................................................... 5 5 SUMMARY IN ENGLISH ....................................................................................................... -
Androctonus Mauretanicus Mauretanicus
Hindawi Publishing Corporation Journal of Toxicology Volume 2012, Article ID 103608, 9 pages doi:10.1155/2012/103608 Review Article Potassium Channels Blockers from the Venom of Androctonus mauretanicus mauretanicus Marie-France Martin-Eauclaire and Pierre E. Bougis Aix-Marseille University, CNRS, UMR 7286, CRN2M, Facult´edeM´edecine secteur Nord, CS80011, Boulevard Pierre Dramard, 13344 Marseille Cedex 15, France Correspondence should be addressed to Marie-France Martin-Eauclaire, [email protected] and Pierre E. Bougis, [email protected] Received 2 February 2012; Accepted 16 March 2012 Academic Editor: Maria Elena de Lima Copyright © 2012 M.-F. Martin-Eauclaire and P. E. Bougis. This is an open access article distributed under the Creative Commons Attribution License, which permits unrestricted use, distribution, and reproduction in any medium, provided the original work is properly cited. K+ channels selectively transport K+ ions across cell membranes and play a key role in regulating the physiology of excitable and nonexcitable cells. Their activation allows the cell to repolarize after action potential firing and reduces excitability, whereas channel inhibition increases excitability. In eukaryotes, the pharmacology and pore topology of several structural classes of K+ channels have been well characterized in the past two decades. This information has come about through the extensive use of scorpion toxins. We have participated in the isolation and in the characterization of several structurally distinct families of scorpion toxin peptides exhibiting different K+ channel blocking functions. In particular, the venom from the Moroccan scorpion Androctonus ffi + + mauretanicus mauretanicus provided several high-a nity blockers selective for diverse K channels (SKCa,Kv4.x, and Kv1.x K channel families). -
Ion Channel-Target Toxicology
Journal of Toxicology Ion Channel-Target Toxicology Guest Editors: Yonghua Ji, Jan Tytgat, Maria Elena de Lima, Hongzhuan Chen, and Yun Zhang Ion Channel-Target Toxicology Journal of Toxicology Ion Channel-Target Toxicology Guest Editors: Yonghua Ji, Jan Tytgat, Maria Elena de Lima, Hongzhuan Chen, and Yun Zhang Copyright © 2012 Hindawi Publishing Corporation. All rights reserved. This is a special issue published in “Journal of Toxicology.” All articles are open access articles distributed under the Creative Commons Attribution License, which permits unrestricted use, distribution, and reproduction in any medium, provided the original work is prop- erly cited. Editorial Board Syed F. Ali, USA Hisato Iwata, Japan Gary H. Perdew, USA Michael Aschner, USA Margaret James, USA Cinta Porte, Spain Thomas Burbacher, USA Yujian James Kang, USA Robert H. Rice, USA Steven J. Bursian, USA Mary Kanz, USA Rudy Richardson, USA James Bus, USA M. Firoze Khan, USA Arleen Rifkind, USA Lucio Guido Costa, USA Paul Kostyniak, USA JeanClare Seagrave, USA Edmond Edmond Creppy, France Robert Krieger, USA James Sikarskie, USA Kevin Crofton, USA Kannan Krishnan, Canada J. J. Stegeman, USA Michael L. Cunningham, USA B. L. Lasley, USA Susan Sumner, USA Anthony DeCaprio, USA Pamela Lein, USA Robert Tanguay, USA David Doolittle, USA Robert Luebke, USA Kenneth Turteltaub, USA Paul R. Ebert, Australia Michael R. Moore, Australia Brad Upham, USA Laurence D. Fechter, USA Jack Ng, Australia William Valentine, USA M. Teresa Colomina Fosch, Spain P. J. O’Brien, Canada J. -
Voltage-Gated Ion Channels Mediate the Electrotaxis of Glioblastoma Cells in a Hybrid PMMA/PDMS Microdevice Hsieh-Fu Tsai,1 Camilo Ijspeert,1 and Amy Q
bioRxiv preprint doi: https://doi.org/10.1101/2020.02.14.948638; this version posted February 20, 2020. The copyright holder for this preprint (which was not certified by peer review) is the author/funder, who has granted bioRxiv a license to display the preprint in perpetuity. It is made available under aCC-BY-NC-ND 4.0 International license. Voltage-gated ion channels mediate the electrotaxis of glioblastoma cells in a hybrid PMMA/PDMS microdevice Hsieh-Fu Tsai,1 Camilo IJspeert,1 and Amy Q. Shen1, a) Micro/Bio/Nanofluidics Unit, Okinawa Institute of Science and Technology Graduate University, Okinawa JAPAN (Dated: 18 February 2020) Transformed astrocytes in the most aggressive form cause glioblastoma, the most common cancer in central nervous system with high mortality. The physiological electric field by neuronal local field potentials and tissue polarity may guide the infiltration of glioblastoma cells through the electrotaxis process. However, microenvironments with multiplex gradients are difficult to create. In this work, we have developed a hybrid microfluidic platform to study glioblastoma electrotaxis in controlled microenvironments with high through- put quantitative analysis by a machine learning-powered single cell tracking software. By equalizing the hydrostatic pressure difference between inlets and outlets of the microchannel, uniform single cells can be seeded reliably inside the microdevice. The electrotaxis of two glioblastoma models, T98G and U-251MG, re- quire optimal laminin-containing extracellular matrix and exhibits opposite directional and electro-alignment tendencies. Calcium signaling is a key contributor in glioblastoma pathophysiology but its role in glioblas- toma electrotaxis is still an open question. Anodal T98G electrotaxis and cathodal U-251MG electrotaxis require the presence of extracellular calcium cations. -
Modulatory Mechanisms and Multiple Functions of Somatodendritic A-Type K+ Channel Auxiliary Subunits
REVIEW ARTICLE published: 27 March 2014 doi: 10.3389/fncel.2014.00082 Modulatory mechanisms and multiple functions of somatodendritic A-type K+ channel auxiliary subunits Henry H. Jerng* and Paul J. Pfaffinger Department of Neuroscience, Baylor College of Medicine, Houston, TX, USA Edited by: Auxiliary subunits are non-conducting, modulatory components of the multi-protein ion Leigh Anne Swayne, University of channel complexes that underlie normal neuronal signaling. They interact with the pore- Victoria, Canada forming α-subunits to modulate surface distribution, ion conductance, and channel gating Reviewed by: properties. For the somatodendritic subthreshold A-type potassium (I ) channel based on Stefan H. Heinemann, SA Friedrich-Schiller-Universität, Germany Kv4 α-subunits, two types of auxiliary subunits have been extensively studied: Kv channel- Douglas A. Bayliss, University of interacting proteins (KChIPs) and dipeptidyl peptidase-like proteins (DPLPs). KChIPs are Virginia, USA cytoplasmic calcium-binding proteins that interact with intracellular portions of the Kv4 *Correspondence: subunits, whereas DPLPs are type II transmembrane proteins that associate with the Kv4 Henry H. Jerng, Department of channel core. Both KChIPs and DPLPs genes contain multiple start sites that are used by Neuroscience, Baylor College of Medicine, One Baylor Plaza, S630, various neuronal populations to drive the differential expression of functionally distinct Houston, TX 77030, USA N-terminal variants. In turn, these N-terminal variants generate tremendous functional e-mail: [email protected] diversity across the nervous system. Here, we focus our review on (1) the molecular mechanism underlying the unique properties of different N-terminal variants, (2) the shaping of native ISA properties by the concerted actions of KChIPs and DPLP variants, and (3) the surprising ways that KChIPs and DPLPs coordinate the activity of multiple channels to fine-tune neuronal excitability. -
Potassium and Sodium Currents Regulating Pacemaking and Burst Firing in Substantia Nigra Dopamine Neurons
Potassium and Sodium Currents Regulating Pacemaking and Burst Firing in Substantia Nigra Dopamine Neurons The Harvard community has made this article openly available. Please share how this access benefits you. Your story matters Citation Kimm, Tilia. 2015. Potassium and Sodium Currents Regulating Pacemaking and Burst Firing in Substantia Nigra Dopamine Neurons. Doctoral dissertation, Harvard University, Graduate School of Arts & Sciences. Citable link http://nrs.harvard.edu/urn-3:HUL.InstRepos:14226104 Terms of Use This article was downloaded from Harvard University’s DASH repository, and is made available under the terms and conditions applicable to Other Posted Material, as set forth at http:// nrs.harvard.edu/urn-3:HUL.InstRepos:dash.current.terms-of- use#LAA Potassium and Sodium Currents Regulating Pacemaking and Burst Firing in Substantia Nigra Dopamine Neurons A dissertation presented by Tilia Akira Noel Kimm to The Division of Medical Sciences in partial fulfillment of the requirements for the degree of Doctor of Philosophy in the subject of Neurobiology Harvard University Cambridge, Massachusetts December 2014 © 2014 Tilia Akira Noel Kimm All rights reserved. Dissertation Advisor: Professor Bruce P. Bean Tilia Akira Noel Kimm Potassium and Sodium Currents Regulating Pacemaking and Burst Firing in Substantia Nigra Dopamine Neurons Abstract Dopamine-releasing neurons with cell bodies in the substantia nigra pars compacta (SNc) are a primary source of dopamine in the mammalian brain. Dysfunction of dopaminergic signaling is associated with numerous psychiatric disorders, and degeneration of the SNc is one of the . Their spontaneous actionhallmarks potentials of Parkinsons supply target disease areas These with neurons baseline are dopaminergic autonomous tone, pacemakers while synaptically-triggered bursts signal salient events.