The RNA Processing Proteins Xrn1 and Rrp6 Regulate DNA Damage Checkpoint Activation and Telomere Metabolism
Total Page:16
File Type:pdf, Size:1020Kb
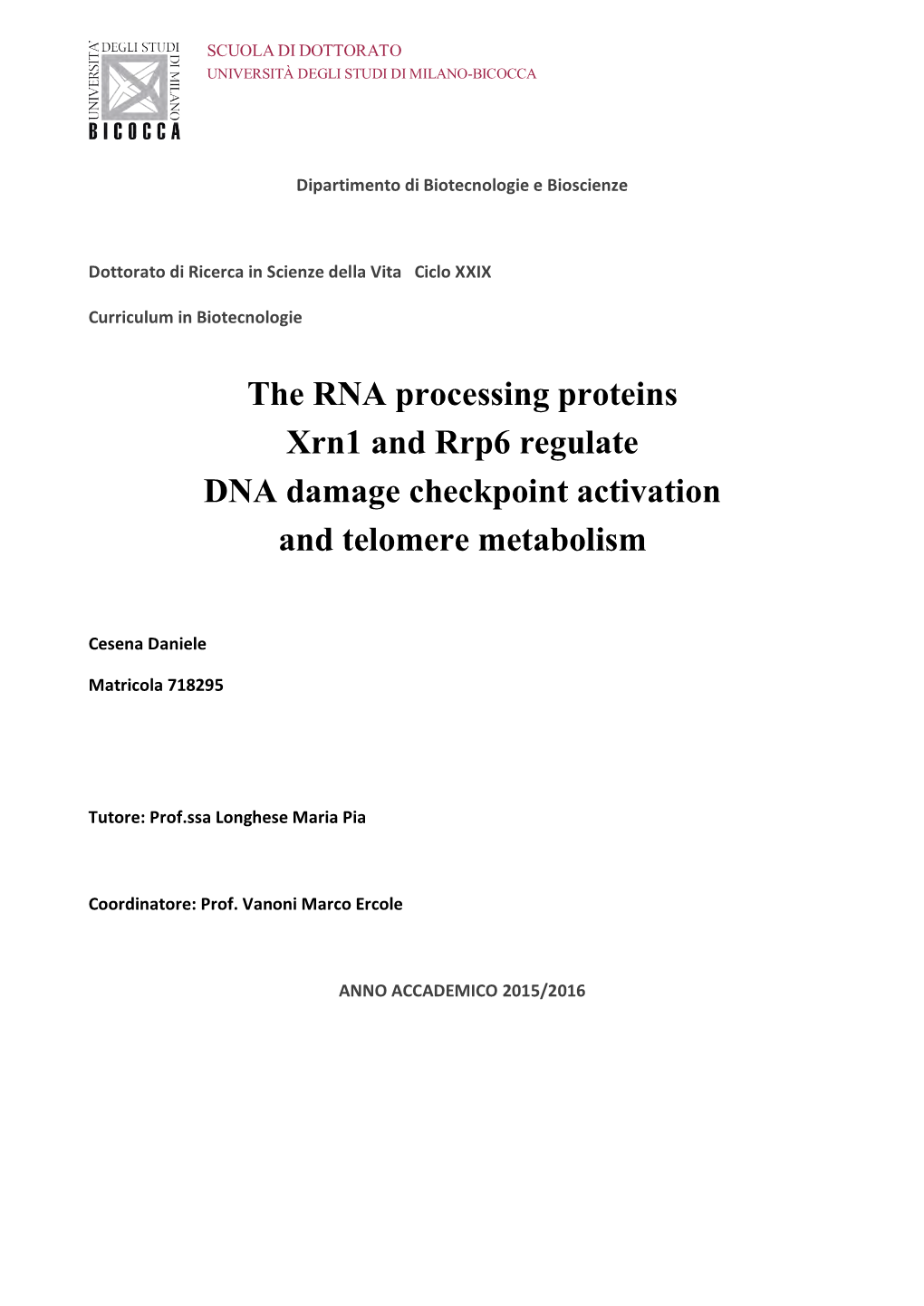
Load more
Recommended publications
-
S41467-020-18249-3.Pdf
ARTICLE https://doi.org/10.1038/s41467-020-18249-3 OPEN Pharmacologically reversible zonation-dependent endothelial cell transcriptomic changes with neurodegenerative disease associations in the aged brain Lei Zhao1,2,17, Zhongqi Li 1,2,17, Joaquim S. L. Vong2,3,17, Xinyi Chen1,2, Hei-Ming Lai1,2,4,5,6, Leo Y. C. Yan1,2, Junzhe Huang1,2, Samuel K. H. Sy1,2,7, Xiaoyu Tian 8, Yu Huang 8, Ho Yin Edwin Chan5,9, Hon-Cheong So6,8, ✉ ✉ Wai-Lung Ng 10, Yamei Tang11, Wei-Jye Lin12,13, Vincent C. T. Mok1,5,6,14,15 &HoKo 1,2,4,5,6,8,14,16 1234567890():,; The molecular signatures of cells in the brain have been revealed in unprecedented detail, yet the ageing-associated genome-wide expression changes that may contribute to neurovas- cular dysfunction in neurodegenerative diseases remain elusive. Here, we report zonation- dependent transcriptomic changes in aged mouse brain endothelial cells (ECs), which pro- minently implicate altered immune/cytokine signaling in ECs of all vascular segments, and functional changes impacting the blood–brain barrier (BBB) and glucose/energy metabolism especially in capillary ECs (capECs). An overrepresentation of Alzheimer disease (AD) GWAS genes is evident among the human orthologs of the differentially expressed genes of aged capECs, while comparative analysis revealed a subset of concordantly downregulated, functionally important genes in human AD brains. Treatment with exenatide, a glucagon-like peptide-1 receptor agonist, strongly reverses aged mouse brain EC transcriptomic changes and BBB leakage, with associated attenuation of microglial priming. We thus revealed tran- scriptomic alterations underlying brain EC ageing that are complex yet pharmacologically reversible. -
Dissertation
Regulation of gene silencing: From microRNA biogenesis to post-translational modifications of TNRC6 complexes DISSERTATION zur Erlangung des DOKTORGRADES DER NATURWISSENSCHAFTEN (Dr. rer. nat.) der Fakultät Biologie und Vorklinische Medizin der Universität Regensburg vorgelegt von Johannes Danner aus Eggenfelden im Jahr 2017 Das Promotionsgesuch wurde eingereicht am: 12.09.2017 Die Arbeit wurde angeleitet von: Prof. Dr. Gunter Meister Johannes Danner Summary ‘From microRNA biogenesis to post-translational modifications of TNRC6 complexes’ summarizes the two main projects, beginning with the influence of specific RNA binding proteins on miRNA biogenesis processes. The fate of the mature miRNA is determined by the incorporation into Argonaute proteins followed by a complex formation with TNRC6 proteins as core molecules of gene silencing complexes. miRNAs are transcribed as stem-loop structured primary transcripts (pri-miRNA) by Pol II. The further nuclear processing is carried out by the microprocessor complex containing the RNase III enzyme Drosha, which cleaves the pri-miRNA to precursor-miRNA (pre-miRNA). After Exportin-5 mediated transport of the pre-miRNA to the cytoplasm, the RNase III enzyme Dicer cleaves off the terminal loop resulting in a 21-24 nt long double-stranded RNA. One of the strands is incorporated in the RNA-induced silencing complex (RISC), where it directly interacts with a member of the Argonaute protein family. The miRNA guides the mature RISC complex to partially complementary target sites on mRNAs leading to gene silencing. During this process TNRC6 proteins interact with Argonaute and recruit additional factors to mediate translational repression and target mRNA destabilization through deadenylation and decapping leading to mRNA decay. -
The Roles of the Exoribonucleases DIS3L2 and XRN1 in Human Disease
View metadata, citation and similar papers at core.ac.uk brought to you by CORE provided by Sussex Research Online The roles of the exoribonucleases DIS3L2 and XRN1 in human disease. Amy L. Pashler1, Ben Towler1, Christopher I. Jones2 and Sarah F. Newbury* 1Brighton and Sussex Medical School, Medical Research building, University of Sussex, Falmer, Brighton, BN1 9PS, U.K. 2Brighton and Sussex Medical School, Department of Primary Care and Public Health, University of Brighton, Falmer, Brighton, BN1 9PH, U.K. *Corresponding author: [email protected] +44 (0)1273 877874 Abstract RNA degradation is a vital post-transcriptional process which ensures that transcripts are maintained at the correct level within the cell. DIS3L2 and XRN1 are conserved exoribonucleases which are critical for the degradation of cytoplasmic RNAs. Although the molecular mechanisms of RNA degradation by DIS3L2 and XRN1 have been well studied, less is known about their specific roles in development of multicellular organisms or human disease. This review focusses on the roles of DIS3L2 and XRN1 in the pathogenesis of human disease, particularly in relation to phenotypes seen in model organisms. The known diseases associated with loss of activity of DIS3L2 and XRN1 are discussed, together with possible mechanisms and cellular pathways leading to these disease conditions. Key words: RNA stability, RNA degradation, human disease, virus-host interactions, XRN1, DIS3L2 Abbreviations used: UTR, untranslated region; HCV, Hepatitis C virus; sfRNA, small flaviviral non- coding RNA; mRNA, messenger RNA; miRNA, microRNA. Introduction Ribonucleases are key enzymes involved in the control of mRNA stability, which is crucially important in maintaining RNA transcripts at the correct levels within cells. -
Xrn1 - a Major Mrna Synthesis and Decay Factor
bioRxiv preprint doi: https://doi.org/10.1101/2021.04.01.437949; this version posted April 1, 2021. The copyright holder for this preprint (which was not certified by peer review) is the author/funder, who has granted bioRxiv a license to display the preprint in perpetuity. It is made available under aCC-BY-NC-ND 4.0 International license. RNA-controlled nucleocytoplasmic shuttling of mRNA decay factors regulates mRNA synthesis and initiates a novel mRNA decay pathway Running title: Cytoplasmic mRNA decay begins in the nucleus Shiladitya Chattopadhyay1, Jose Garcia-Martinez2, Gal Haimovich1,3, Aya Khwaja1, Oren Barkai1, Ambarnil Ghosh4, Silvia Gabriela Chuarzman5, Maya Schuldiner5, Ron Elran1, Miriam Rosenberg1, Katherine Bohnsack6, Markus Bohnsack6, Jose E Perez-Ortin2, Mordechai Choder1* 1Department of Molecular Microbiology, Rappaport Faculty of Medicine, Technion-Israel Institute of Technology, Haifa 31096, Israel. 2Instituto de Biotecnología y Biomedicina (Biotecmed), Universitat de València ; Burjassot, Valencia, 46100, Spain. 3current address:Department of Molecular Genetics, Weizmann Institute of Science, Rehovot 7610001, Israel. 4UCD Conway Institute of Biomolecular & Biomedical Research, Dublin, Ireland. 5Department of Molecular Genetics, Weizmann Institute of Science, Rehovot 7610001, Israel 6Institute for Molecular Biology, University Medical Center Göttingen Georg-August- University, Göttingen, Germany Göttingen Centre for Molecular Biosciences, Georg-August- University, Göttingen, Germany *Corresponding Author: [email protected] bioRxiv preprint doi: https://doi.org/10.1101/2021.04.01.437949; this version posted April 1, 2021. The copyright holder for this preprint (which was not certified by peer review) is the author/funder, who has granted bioRxiv a license to display the preprint in perpetuity. It is made available under aCC-BY-NC-ND 4.0 International license. -
Effect of Cigarette Smoke on DNA Methylation and Lung Function
de Vries et al. Respiratory Research (2018) 19:212 https://doi.org/10.1186/s12931-018-0904-y RESEARCH Open Access From blood to lung tissue: effect of cigarette smoke on DNA methylation and lung function Maaike de Vries1,2* , Diana A van der Plaat1,2, Ivana Nedeljkovic3, Rikst Nynke Verkaik-Schakel4, Wierd Kooistra2,5, Najaf Amin3, Cornelia M van Duijn3, Corry-Anke Brandsma2,5, Cleo C van Diemen6, Judith M Vonk1,2 and H Marike Boezen1,2 Abstract Background: Genetic and environmental factors play a role in the development of COPD. The epigenome, and more specifically DNA methylation, is recognized as important link between these factors. We postulate that DNA methylation is one of the routes by which cigarette smoke influences the development of COPD. In this study, we aim to identify CpG-sites that are associated with cigarette smoke exposure and lung function levels in whole blood and validate these CpG-sites in lung tissue. Methods: The association between pack years and DNA methylation was studied genome-wide in 658 current smokers with >5 pack years using robust linear regression analysis. Using mediation analysis, we subsequently selected the CpG-sites that were also associated with lung function levels. Significant CpG-sites were validated in lung tissue with pyrosequencing and expression quantitative trait methylation (eQTM) analysis was performed to investigate the association between DNA methylation and gene expression. Results: 15 CpG-sites were significantly associated with pack years and 10 of these were additionally associated with lung function levels. We validated 5 CpG-sites in lung tissue and found several associations between DNA methylation and gene expression. -
Genome-Wide Analyses of XRN1-Sensitive Targets in Osteosarcoma Cells Identifies Disease-Relevant 2 Transcripts Containing G-Rich Motifs
Downloaded from rnajournal.cshlp.org on October 11, 2021 - Published by Cold Spring Harbor Laboratory Press 1 Genome-wide analyses of XRN1-sensitive targets in osteosarcoma cells identifies disease-relevant 2 transcripts containing G-rich motifs. 3 Amy L. Pashler, Benjamin P. Towler+, Christopher I. Jones, Hope J. Haime, Tom Burgess, and Sarah F. 4 Newbury1+ 5 6 Brighton and Sussex Medical School, University of Sussex, Brighton, BN1 9PS, UK 7 8 +Corresponding author: Prof Sarah Newbury, Medical Research Building, Brighton and Sussex 9 Medical School, University of Sussex, Falmer, Brighton BN1 9PS, UK. 10 Tel: +44(0)1273 877874 11 [email protected] 12 13 +Co-corresponding author: Dr Ben Towler, Medical Research Building, Brighton and Sussex Medical 14 School, University of Sussex, Falmer, Brighton BN1 9PS, UK. 15 Tel: +44(0)1273 877876 16 [email protected] 17 18 Running title: Genome-wide analyses of XRN1 targets in OS cells 19 20 Key words: XRN1, RNA-seq, Ewing sarcoma, lncRNAs, RNA degradation 21 22 23 24 25 26 27 28 29 30 31 32 33 Pashler et al 1 Downloaded from rnajournal.cshlp.org on October 11, 2021 - Published by Cold Spring Harbor Laboratory Press 34 35 ABSTRACT 36 XRN1 is a highly conserved exoribonuclease which degrades uncapped RNAs in a 5’-3’ direction. 37 Degradation of RNAs by XRN1 is important in many cellular and developmental processes and is 38 relevant to human disease. Studies in D. melanogaster demonstrate that XRN1 can target specific 39 RNAs, which have important consequences for developmental pathways. -
Differential Expression Profile Prioritization of Positional Candidate Glaucoma Genes the GLC1C Locus
LABORATORY SCIENCES Differential Expression Profile Prioritization of Positional Candidate Glaucoma Genes The GLC1C Locus Frank W. Rozsa, PhD; Kathleen M. Scott, BS; Hemant Pawar, PhD; John R. Samples, MD; Mary K. Wirtz, PhD; Julia E. Richards, PhD Objectives: To develop and apply a model for priori- est because of moderate expression and changes in tization of candidate glaucoma genes. expression. Transcription factor ZBTB38 emerges as an interesting candidate gene because of the overall expres- Methods: This Affymetrix GeneChip (Affymetrix, Santa sion level, differential expression, and function. Clara, Calif) study of gene expression in primary cul- ture human trabecular meshwork cells uses a positional Conclusions: Only1geneintheGLC1C interval fits our differential expression profile model for prioritization of model for differential expression under multiple glau- candidate genes within the GLC1C genetic inclusion in- coma risk conditions. The use of multiple prioritization terval. models resulted in filtering 7 candidate genes of higher interest out of the 41 known genes in the region. Results: Sixteen genes were expressed under all condi- tions within the GLC1C interval. TMEM22 was the only Clinical Relevance: This study identified a small sub- gene within the interval with differential expression in set of genes that are most likely to harbor mutations that the same direction under both conditions tested. Two cause glaucoma linked to GLC1C. genes, ATP1B3 and COPB2, are of interest in the con- text of a protein-misfolding model for candidate selec- tion. SLC25A36, PCCB, and FNDC6 are of lesser inter- Arch Ophthalmol. 2007;125:117-127 IGH PREVALENCE AND PO- identification of additional GLC1C fami- tential for severe out- lies7,18-20 who provide optimal samples for come combine to make screening candidate genes for muta- adult-onset primary tions.7,18,20 The existence of 2 distinct open-angle glaucoma GLC1C haplotypes suggests that muta- (POAG) a significant public health prob- tions will not be limited to rare descen- H1 lem. -
Exoribonuclease Xrn1 by Cofactor Dcs1 Is Essential for Mitochondrial Function in Yeast
Activation of 5′-3′ exoribonuclease Xrn1 by cofactor Dcs1 is essential for mitochondrial function in yeast Flore Sinturela,b, Dominique Bréchemier-Baeyb, Megerditch Kiledjianc, Ciarán Condonb, and Lionel Bénarda,b,1 aLaboratoire de Biologie Moléculaire et Cellulaire des Eucaryotes, Institut de Biologie Physico-Chimique, Centre National de la Recherche Scientifique, Formation de Recherche en Evolution (FRE) 3354, Université Pierre et Marie Curie, 75005 Paris, France; bLaboratoire de l’Expression Génétique Microbienne, Institut de Biologie Physico-Chimique, Centre National de la Recherche Scientifique, Unité Propre de Recherche 9073, Université de Paris 7-Denis-Diderot, 75005 Paris, France; and cDepartment of Cell Biology and Neuroscience, Rutgers University, Piscataway, NJ 08854 Edited* by Reed B. Wickner, National Institutes of Health, Bethesda, MD, and approved April 6, 2012 (received for review December 6, 2011) The scavenger decapping enzyme Dcs1 has been shown to facilitate Saccharomyces cerevisiae (16). Known regulatory RNAs belong to the activity of the cytoplasmic 5′-3′ exoribonuclease Xrn1 in eukar- this class, and their stabilization in the absence of Xrn1 could yotes. Dcs1 has also been shown to be required for growth in glyc- explain the aberrant expression of some genes. erol medium. We therefore wondered whether the capacity to Few studies have investigated how the activity of exoribonu- activate RNA degradation could account for its requirement for cleases is modulated in connection to cell physiology (17, 18). growth on this carbon source. Indeed, a catalytic mutant of Xrn1 Here, we focus on Dcs1, a factor that potentially controls the is also unable to grow in glycerol medium, and removal of the activity of the exoribonuclease Xrn1 (4). -
3′ Exoribonuclease 2 As a Potential Target for Developing Fungicides To
atholog P y & nt a M Bonilla and Calderón-Oropeza, J Plant Pathol Microbiol 2018, 9:9 l i P c f r o o b DOI: 10.4172/2157-7471.1000453 l i Journal of a o l n o r g u y o J ISSN: 2157-7471 Plant Pathology & Microbiology ReviewResearch Article Article OpenOpen Access Access The 5´ → 3´ Exoribonuclease 2 as a Potential Target for Developing Fungicides to Control the Panama Disease Maldonado Bonilla LD* and Calderón-Oropeza MA Institute of Genetics, Universidad del Mar Campus Puerto Escondido, Mexico Abstract An outbreak of Fusarium oxysporum f. sp. cubense Tropical Race 4 is currently threatening the global production of bananas. Due to the clonal nature of commercial banana plants, selecting resistant cultivars does not seem feasible; therefore, alternative approaches to crop protection must be developed. The 5´ → 3´ exoribonuclease XRN2/RAT1 is involved in 5´ → 3´ RNA decay. Fungal studies with XRN2 and conditional mutants have illustrated the crucial role of this enzyme, suggesting XRN2 should be considered as target to searching for novel inhibitors that might be used as fungicides to control Panama disease. Our in silico analysis of Tropical Race 4 XRN2 (FocTR4XRN2) revealed characteristic features of 5´ → 3´ exoribonuclease such as the catalytic domain that recognizes 5´-monophosphorylated RNA and catalyses the processed cleavage of mononucleotides. A delimited cavity showing the potential for substrate uptake appears prone to interacting with small molecules that might inhibit its activity. The catalytic domain in FocTR4XRN2 harbors a CCHC motif, which is conserved in orthologous proteins from filamentous fungi but lacking in yeasts. -
Antiviral Rnai in Insects and Mammals: Parallels and Differences
viruses Review Antiviral RNAi in Insects and Mammals: Parallels and Differences Susan Schuster, Pascal Miesen and Ronald P. van Rij * Department of Medical Microbiology, Radboud University Medical Center, Radboud Institute for Molecular Life Sciences, 6500 HB Nijmegen, The Netherlands; [email protected] (S.S.); [email protected] (P.M.) * Correspondence: [email protected]; Tel.: +31-24-3617574 Received: 16 April 2019; Accepted: 15 May 2019; Published: 16 May 2019 Abstract: The RNA interference (RNAi) pathway is a potent antiviral defense mechanism in plants and invertebrates, in response to which viruses evolved suppressors of RNAi. In mammals, the first line of defense is mediated by the type I interferon system (IFN); however, the degree to which RNAi contributes to antiviral defense is still not completely understood. Recent work suggests that antiviral RNAi is active in undifferentiated stem cells and that antiviral RNAi can be uncovered in differentiated cells in which the IFN system is inactive or in infections with viruses lacking putative viral suppressors of RNAi. In this review, we describe the mechanism of RNAi and its antiviral functions in insects and mammals. We draw parallels and highlight differences between (antiviral) RNAi in these classes of animals and discuss open questions for future research. Keywords: small interfering RNA; RNA interference; RNA virus; antiviral defense; innate immunity; interferon; stem cells 1. Introduction RNA interference (RNAi) or RNA silencing was first described in the model organism Caenorhabditis elegans [1] and following this ground-breaking discovery, studies in the field of small, noncoding RNAs have advanced tremendously. RNAi acts, with variations, in all eukaryotes ranging from unicellular organisms to complex species from the plant and animal kingdoms [2]. -
High-Throughput Sirna Screening Reveals Functional Interactions and Redundancies Of
bioRxiv preprint doi: https://doi.org/10.1101/2020.08.05.238006; this version posted August 6, 2020. The copyright holder for this preprint (which was not certified by peer review) is the author/funder, who has granted bioRxiv a license to display the preprint in perpetuity. It is made available under aCC-BY-NC-ND 4.0 International license. High-throughput siRNA screening reveals functional interactions and redundancies of human processive exoribonucleases Anna Hojka-Osinska1,2, ‡, Aleksander Chlebowski2,‡, Ewelina P. Owczarek2, Kamila Afek2, Kamila Kłosowska2, Roman J. Szczesny2#, Andrzej Dziembowski1,2,3# 1 International Institute of Molecular and Cell Biology, 02-109, Warsaw, Poland 2 Institute of Biochemistry and Biophysics Polish Academy of Sciences, 02-106, Warsaw, Poland 3Department of Genetics and Biotechnology, Faculty of Biology, University of Warsaw, 02- 096, Warsaw, Poland ‡These authors contributed equally to this work *Corresponding author 1 bioRxiv preprint doi: https://doi.org/10.1101/2020.08.05.238006; this version posted August 6, 2020. The copyright holder for this preprint (which was not certified by peer review) is the author/funder, who has granted bioRxiv a license to display the preprint in perpetuity. It is made available under aCC-BY-NC-ND 4.0 International license. ABSTRACT Processive exoribonucleases, the executors of RNA decay, participate in multiple physical and functional interactions. Unlike physical ones, functional relationships have not been investigated in human cells. Here we have screened cells deficient in DIS3, XRN2, EXOSC10, DIS3L, and DIS3L2 with a custom siRNA library and determined their functional interactions with diverse pathways of RNA metabolism. -
Exoribonuclease Xrn1 by Cofactor Dcs1 Is Essential for Mitochondrial Function in Yeast
Activation of 5′-3′ exoribonuclease Xrn1 by cofactor Dcs1 is essential for mitochondrial function in yeast Flore Sinturela,b, Dominique Bréchemier-Baeyb, Megerditch Kiledjianc, Ciarán Condonb, and Lionel Bénarda,b,1 aLaboratoire de Biologie Moléculaire et Cellulaire des Eucaryotes, Institut de Biologie Physico-Chimique, Centre National de la Recherche Scientifique, Formation de Recherche en Evolution (FRE) 3354, Université Pierre et Marie Curie, 75005 Paris, France; bLaboratoire de l’Expression Génétique Microbienne, Institut de Biologie Physico-Chimique, Centre National de la Recherche Scientifique, Unité Propre de Recherche 9073, Université de Paris 7-Denis-Diderot, 75005 Paris, France; and cDepartment of Cell Biology and Neuroscience, Rutgers University, Piscataway, NJ 08854 Edited* by Reed B. Wickner, National Institutes of Health, Bethesda, MD, and approved April 6, 2012 (received for review December 6, 2011) The scavenger decapping enzyme Dcs1 has been shown to facilitate Saccharomyces cerevisiae (16). Known regulatory RNAs belong to the activity of the cytoplasmic 5′-3′ exoribonuclease Xrn1 in eukar- this class, and their stabilization in the absence of Xrn1 could yotes. Dcs1 has also been shown to be required for growth in glyc- explain the aberrant expression of some genes. erol medium. We therefore wondered whether the capacity to Few studies have investigated how the activity of exoribonu- activate RNA degradation could account for its requirement for cleases is modulated in connection to cell physiology (17, 18). growth on this carbon source. Indeed, a catalytic mutant of Xrn1 Here, we focus on Dcs1, a factor that potentially controls the is also unable to grow in glycerol medium, and removal of the activity of the exoribonuclease Xrn1 (4).