Quantum Dots
Total Page:16
File Type:pdf, Size:1020Kb
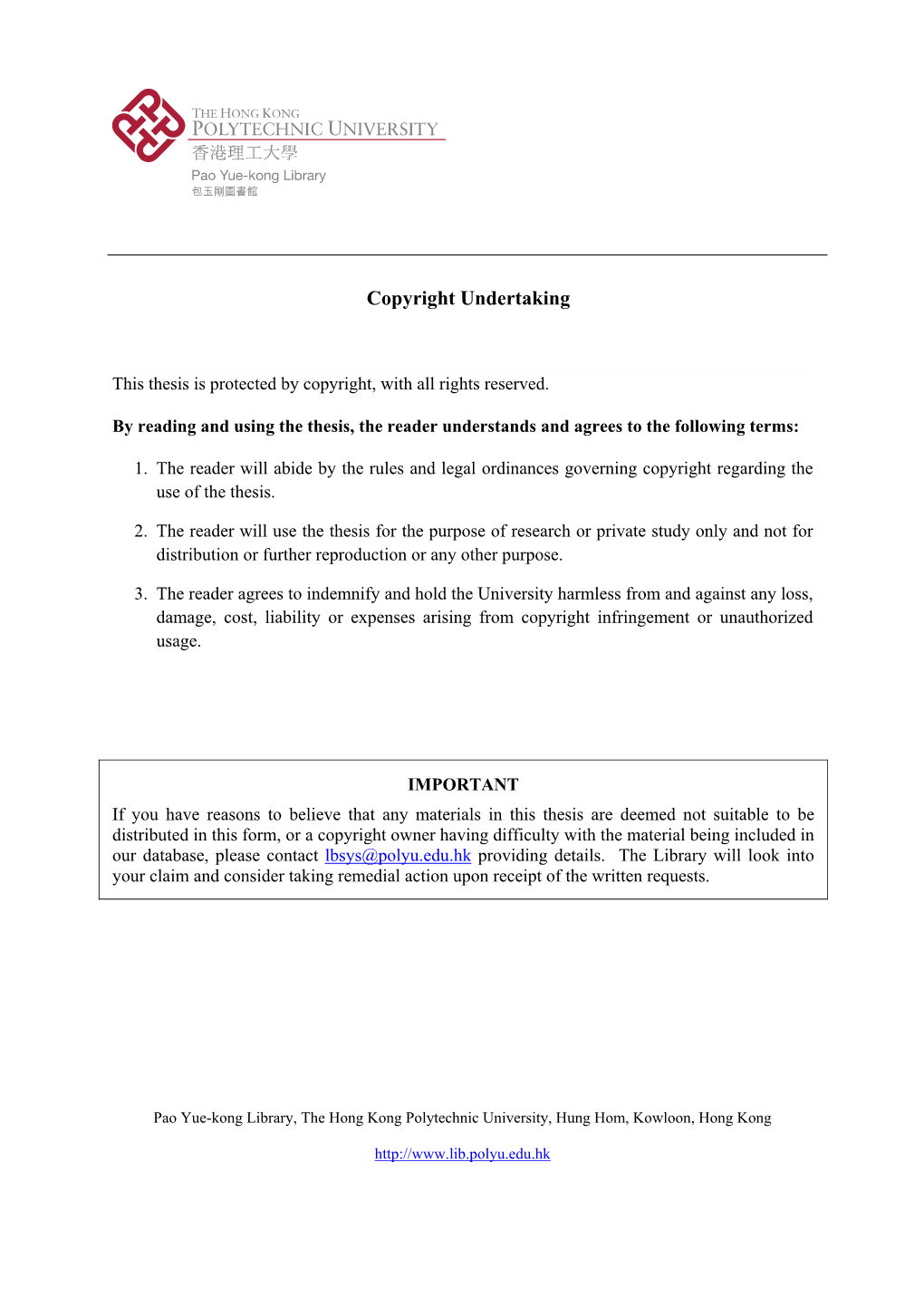
Load more
Recommended publications
-
Wide Spectral Photoresponse of Layered Platinum Diselenide-Based Photodiodes † ‡ § ∥ † ⊥ † # Chanyoung Yim, , Niall Mcevoy, , Sarah Riazimehr, , Daniel S
This is an open access article published under an ACS AuthorChoice License, which permits copying and redistribution of the article or any adaptations for non-commercial purposes. Letter Cite This: Nano Lett. 2018, 18, 1794−1800 pubs.acs.org/NanoLett Wide Spectral Photoresponse of Layered Platinum Diselenide-Based Photodiodes † ‡ § ∥ † ⊥ † # Chanyoung Yim, , Niall McEvoy, , Sarah Riazimehr, , Daniel S. Schneider, Farzan Gity, # # ∇ † ⊥ ○ ‡ § ∥ Scott Monaghan, Paul K. Hurley, , Max C. Lemme,*, , , and Georg S. Duesberg*, , , † Department of Electrical Engineering and Computer Science, University of Siegen, Hölderlinstraße 3, 57076 Siegen, Germany ‡ Institute of Physics, EIT 2, Faculty of Electrical Engineering and Information Technology, Universitaẗ der Bundeswehr München, Werner-Heisenberg-Weg 39, 85577 Neubiberg, Germany § School of Chemistry, Trinity College Dublin, Dublin 2, Ireland ∥ Centre for the Research on Adaptive Nanostructures and Nanodevices (CRANN) and Advanced Materials and BioEngineering Research (AMBER), Trinity College Dublin, Dublin 2, Ireland ⊥ Chair of Electronic Devices, Faculty of Electrical Engineering and Information Technology, RWTH Aachen University, Otto-Blumenthal-Str. 2, 52074 Aachen, Germany # Tyndall National Institute, University College Cork, Lee Maltings, Dyke Parade, Cork T12 R5CP, Ireland ∇ Department of Chemistry, University College Cork, Lee Maltings, Dyke Parade, Cork T12 R5CP, Ireland ○ AMO GmbH, Advanced Microelectronic Center Aachen, Otto-Blumenthal-Str. 25, 52074 Aachen, Germany *S Supporting Information ABSTRACT: Platinum diselenide (PtSe2) is a group-10 transition metal dichalcogenide (TMD) that has unique electronic properties, in particular a semimetal-to-semiconductor transition when going from bulk to monolayer form. We report on vertical hybrid Schottky fi barrier diodes (SBDs) of two-dimensional (2D) PtSe2 thin lms on crystalline n-type silicon. -
Atomic-Layer Controlled Interfacial Band Engineering at Two
Article www.acsnano.org Atomic-Layer Controlled Interfacial Band Engineering at Two-Dimensional Layered ffi PtSe2/Si Heterojunctions for E cient Photoelectrochemical Hydrogen Production △ △ Cheng-Chu Chung, Han Yeh, Po-Hsien Wu, Cheng-Chieh Lin, Chia-Shuo Li, Tien-Tien Yeh, Yi Chou, Chuan-Yu Wei, Cheng-Yen Wen, Yi-Chia Chou, Chih-Wei Luo, Chih-I Wu, Ming-Yang Li, Lain-Jong Li, Wen-Hao Chang,* and Chun-Wei Chen* Cite This: https://dx.doi.org/10.1021/acsnano.0c08970 Read Online ACCESS Metrics & More Article Recommendations *sı Supporting Information ABSTRACT: Platinum diselenide (PtSe2) is a group-10 two- dimensional (2D) transition metal dichalcogenide that exhibits the most prominent atomic-layer-dependent electronic behav- ior of “semiconductor-to-semimetal” transition when going from monolayer to bulk form. This work demonstrates an efficient photoelectrochemical (PEC) conversion for direct solar-to-hydrogen (H2) production based on 2D layered PtSe2/ Si heterojunction photocathodes. By systematically controlling fi the number of atomic layers of wafer-scale 2D PtSe2 lms through chemical vapor deposition (CVD), the interfacial band alignments at the 2D layered PtSe2/Si heterojunctions can be p- fi appropriately engineered. The 2D PtSe2/ Si heterojunction photocathode consisting of a PtSe2 thin lm with a thickness of 2.2 nm (or 3 atomic layers) exhibits the optimized band alignment and delivers the best PEC performance for hydrogen production with a photocurrent density of −32.4 mA cm−2 at 0 V and an onset potential of 1 mA cm−2 at 0.29 V versus a reversible hydrogen electrode (RHE) after post-treatment. -
High Carrier Mobility Epitaxially Aligned Ptse2 Films Grown by One-Zone Selenization
ACCEPTED MANUSCRIPT Final published version of this article: Applied Surface Science, Available online 22 September 2020, 147936 DOI: https://doi.org/10.1016/j.apsusc.2020.147936 © 2020. This manuscript version is made available under the CC-BY-NC-ND 4.0 license http://creativecommons.org/licenses/by-nc-nd/4.0/ High carrier mobility epitaxially aligned PtSe2 films grown by one-zone selenization Michaela Sojková1*, Edmund Dobročka1, Peter Hutár1, Valéria Tašková1, Lenka Pribusová- Slušná1, Roman Stoklas1, Igor Píš2, Federica Bondino2, Frans Munnik3 and Martin Hulman1 1Institute of Electrical Engineering, SAS, Dúbravská cesta 9, 841 04 Bratislava, Slovakia 2IOM-CNR, Laboratorio TASC, S.S. 14 km 163.5, 34149 Basovizza, Trieste, Italy 3Helmholtz-ZentrumDresden-Rossendorf, e.V. Bautzner Landstrasse 400, D-01328 Dresden, Germany Corresponding author Email: [email protected] KEYWORDS: PtSe2, epitaxial films, Laue oscillations, Raman spectroscopy 1 ABSTRACT: Few-layer PtSe2 films are promising candidates for applications in high-speed electronics, spintronics and photodetectors. Reproducible fabrication of large-area highly crystalline films is, however, still a challenge. Here, we report the fabrication of epitaxially aligned PtSe2 films using one-zone selenization of pre-sputtered platinum layers. We have studied the influence of growth conditions on structural and electrical properties of the films prepared from Pt layers with different initial thickness. The best results were obtained for the PtSe2 layers grown at elevated temperatures (600 °C). The films exhibit signatures for a long- range in-plane ordering resembling an epitaxial growth. The charge carrier mobility determined by Hall-effect measurements is up to 24 cm2/V.s. 1. -
Two-Dimensional Materials for Infrared Detection
Two-Dimensional Materials for Infrared Detection George Zhang Electrical Engineering and Computer Sciences University of California, Berkeley Technical Report No. UCB/EECS-2021-12 http://www2.eecs.berkeley.edu/Pubs/TechRpts/2021/EECS-2021-12.html May 1, 2021 Copyright © 2021, by the author(s). All rights reserved. Permission to make digital or hard copies of all or part of this work for personal or classroom use is granted without fee provided that copies are not made or distributed for profit or commercial advantage and that copies bear this notice and the full citation on the first page. To copy otherwise, to republish, to post on servers or to redistribute to lists, requires prior specific permission. Acknowledgement First, I would like to thank my advisor, Professor Ali Javey, for the rare opportunity to research among his prolific team and for his strong support and guidance throughout my graduate career. Second, I would like to thank Dr. Matin Amani, Dr. Chaoliang Tan, and Professor James Bullock for their constant mentorship. Third, I would like to thank Professor Vivek Subramanian, Professor Eli Yablonovitch, and Professor Ana Arias for sharing their research passions through excellent teaching. Finally, I would like to thank my family for all of the sacrifices they have made for me. Scanned by CamScanner Acknowledgements First, I would like to thank my advisor, Professor Ali Javey, for the rare opportunity to research among his prolific team and for his strong support and guidance throughout my graduate career. Second, I would like to thank Dr. Matin Amani, Dr. Chaoliang Tan, and Professor James Bullock for their constant mentorship. -
Abstract Book
ABSTRACT BOOK th The 8 International Conference on Nanomaterials and Advanced Energy Storage Systems (INESS-2020) www.iness.kz 6 August, 2020 | Nur-Sultan, Kazakhstan 1 INESS-2020 The 8th International Conference on Nanomaterials and Advanced Energy Storage Systems (INESS-2020) 2 INESS-2020 The 8th International Conference on Nanomaterials and Advanced Energy Storage Systems (INESS-2020) Dear Colleagues! We greatly appreciate your participation and valuable contribution to our Conference. We are honored and pleased to welcome you at INESS-2020! The Organizers will put all efforts to make this day at INESS very efficient time to exchange and discuss the ideas, establish and strengthen collaboration in various fields of research. We hope that INESS will serve as an effective platform to establish new opportunities for joint works in science and education for sustainable development and the best future. We will be looking forward to seeing you again. Yours sincerely, On behalf of the Organizers, Prof. Zhumabay Bakenov ORGANIZERS Nazarbayev University Institute of Batteries LLC National Laboratory Astana (NLA) INESS-2020 3 The 8th International Conference on Nanomaterials and Advanced Energy Storage Systems (INESS-2020) ORGANIZING COMMITTEE Position - Name Organization Chairman - Prof. Ilesanmi Adesida Nazarbayev University, Kazakhstan Co-Chairman - Vassilios Tourassis Nazarbayev University, Kazakhstan Co-Chairman - Prof. Zhaxybay Zhumadilov National Laboratory Astana, Kazakhstan Co-Chairman - Prof. Zhumabay Bakenov Institute of Batteries LLP, National Laboratory Astana, Nazarbayev University, Kazakhstan Member - Prof. Almagul Mentbayeva Nazarbayev University, Kazakhstan Member - Dr. Indira Kurmanbayeva NLA, Nazarbayev University, Kazakhstan Member - Dr. Arailym Nurpeissova NLA, Nazarbayev University, Kazakhstan Member - Dr. Berik Uzakbaiuly NLA, Nazarbayev University, Kazakhstan Member - Dr. -
Structure Characterization and Electronic Properties Investigation Of
Structure Characterization and Electronic Properties Investigation of Two-Dimensional Materials Fazel Baniasadi Dissertation submitted to the faculty of the Virginia Polytechnic Institute and State University in partial fulfillment of the requirements for the degree of Doctor of Philosophy Chair: Chenggang Tao Co-Chair: Kyungwha Park William Reynolds Mitsu Murayama Carlos Suchicital 05/10/2021 Blacksburg, Virginia Keywords: Two Dimensional Materials, Scanning Tunneling Microscopy, Density Functional Theory, Raman Spectroscopy, 1T-PtSe2, 2M-WS2 Copyright (optional – © or Creative Commons, see last page of template for information) Structure and Electronic Properties Investigation of Two-Dimensional Material Fazel Baniasadi ABSTRACT This dissertation will have three chapters. In chapter one, a comprehensive review on defects in two dimensional materials will be presented. The aim of this review is to elaborate on different types of defects in two dimensional (2D) materials like graphene and transition metal dichalcogenides (TMDs). First, different types of point and line defects, e.g. vacancies, anti-sites, guest elements, adatoms, vacancy clusters, grain boundaries, and edges, in these materials are categorized in terms of structure. Second, interactions among defects are discussed in terms of their rearrangement for low-energy configurations. Before studying the electronic and magnetic properties of defective 2D materials, some of the structures are considered in order to see how defect structure evolves to a stable defect configuration. Next, the influence of defects on electronic and magnetic properties of 2D materials is discussed. Finally, the dynamic behavior of defects and 2D structures under conditions such as electron beam irradiation, heat treatment, and ambient conditions, is discussed. Later as a case study, defects in a two dimensional transition metal dichalcogenide will be presented. -
Arxiv:2010.13985V2 [Cond-Mat.Mtrl-Sci] 28 Mar 2021
Defect-induced 4p-magnetism in layered platinum diselenide Priyanka Manchanda, Pankaj Kumar, and Pratibha Dev Department of Physics and Astronomy, Howard University, Washington, D.C. 20059, USA Platinum diselenide (PtSe2) is a recently-discovered extrinsic magnet, with its magnetism at- tributed to the presence of Pt-vacancies. The host material to these defects itself displays interest- ing structural and electronic properties, some of which stem from an unusually strong interaction between its layers. To date, it is not clear how the unique intrinsic properties of PtSe2 will affect its induced magnetism. In this theoretical work, we show that the defect-induced magnetism in PtSe2 thin films is highly sensitive to: (i) defect density, (ii) strain, (iii) layer-thickness, and (iv) substrate choice. These different factors dramatically modify all magnetic properties, including the magnitude of local moments, strength of the coupling, and even nature of the coupling between the moments. We further show that the strong inter-layer interactions are key to understanding these effects. A better understanding of the various influences on magnetism can enable controllable tuning of the magnetic properties in Pt-based dichalcogenides, which can be used to design novel devices for magnetoelectric and magneto-optic applications. I. INTRODUCTION ical works have proposed other strategies for inducing a magnetic moment in PtSe2 monolayers, such as: (i) com- bining strain with hole doping to induce magnetism21, The recent discovery of magnetism in two dimensional (ii) Se-vacancy in the simultaneous presence of strain22, 1{4 (2D) layered materials has sparked renewed interest (iii) hydrogenation on Se sites23, and (iv) doping with in one of the oldest and most well-studied emergent transition metal elements24. -
Volume 64, Issue 2, April 2020 Published by Johnson Matthey © Copyright 2020 Johnson Matthey
ISSN 2056-5135 Johnson Matthey’s international journal of research exploring science and technology in industrial applications Volume 64, Issue 2, April 2020 Published by Johnson Matthey www.technology.matthey.com © Copyright 2020 Johnson Matthey Johnson Matthey Technology Review is published by Johnson Matthey Plc. This work is licensed under a Creative Commons Attribution-NonCommercial-NoDerivatives 4.0 International License. You may share, copy and redistribute the material in any medium or format for any lawful purpose. You must give appropriate credit to the author and publisher. You may not use the material for commercial purposes without prior permission. You may not distribute modifi ed material without prior permission. The rights of users under exceptions and limitations, such as fair use and fair dealing, are not aff ected by the CC licenses. www.technology.matthey.com www.technology.matthey.com Johnson Matthey’s international journal of research exploring science and technology in industrial applications Contents Volume 64, Issue 2, April 2020 101 Guest Editorial: The Importance of Interdisciplinary Science: When Chemistry Needs Physics By Andrew Smith 103 Ab initio Structure Prediction Methods for Battery Materials By Angela F. Harper, Matthew L. Evans, James P. Darby, Bora Karasulu, Can P. Koçer, Joseph R. Nelson and Andrew J. Morris 119 Autothermal Fixed Bed Updraft Gasification of Olive Pomace Biomass and Renewable Energy Generation via Organic Rankine Cycle Turbine By Murat Dogru and Ahmet Erdem 135 In the Lab: Targeting Industry-Compatible Synthesis of Two-Dimensional Materials Featuring Niall McEvoy 138 Plasma Catalysis: A Review of the Interdisciplinary Challenges Faced By Peter Hinde, Vladimir Demidyuk, Alkis Gkelios and Carl Tipton 148 Nanosurfaces 2019 A conference review by Alistair Kean and Sara Coles 152 Observing Solvent Dynamics in Porous Carbons by Nuclear Magnetic Resonance By Luca Cervini, Nathan Barrow and John Griffin 165 Insights into Automotive Particulate Filters using Magnetic Resonance Imaging By J. -
Recent Publications – Faculty of Engineering, Mathematics and Science
Recent Publications – Faculty of Engineering, Mathematics and Science BY MEMBERS OF THE STAFF AND BY RESEARCH STUDENTS WORKING UNDER THEIR SUPERVISION The publications information has been derived from the College’s Research Support System. Every effort has been made to ensure that the information is accurate and complete. Please notify the Research Support Systems Administrator (email: [email protected]) of any errors or omissions which will be corrected in next year’s Calendar. School of Biochemistry and Immunology BIOCHEMISTRY Budanov, Andrei, ‘Distinct role of Sesn2 in response to UVB-induced DNA damage and UVA- induced oxidative stress in melanocytes’, Photochemistry and Photobiology, 93, 1 (2017), 375- 381 [B. Zhao, P. Shah, L. Qiang, T.C. He, A. Budanov, Y.Y. He] ‘Sestrin2 is significantly increased in malignant pleural effusions due to lung cancer and is potentially secreted by pleural mesothelial cells’, Clinical Biochemistry, 49, 9 (2016), 726-728 [I. Tsilioni, A.S. Filippidis, T. Kerenidi, A. Budanov, S.G. Zarogiannis, K.I. Gourgoulianis] ‘Sestrin2 promotes LKB1-mediated AMPK activation in the ischemic heart’, FASEB Journal, 29, 2 (2015), 408-417 [A. Morrison, L. Chen, J. Wang, M. Zhang, H. Yang, Y. Ma, A. Budanov, J.H. Lee, M. Karin, J. Li] ‘Sestrins regulate mTORC1 via RRAGs: the riddle of GATOR’, Molecular and Cellular Oncology, 2, 3 (2015), 3 ‘Sensing the environment through sestrins: implications for cellular metabolism’, International review of cell and molecular biology, eds Kwan W. Jeon and Lorenzo Galluzzi, Elsevier (2016), 1-24 [A. Parmigiani, A. Budanov] ‘Sestrin2 is induced by glucose starvation via the unfolded protein response and protects cells from non-canonical necroptotic cell death’, Scientific Reports, 6, 22538 (2016), 14 [B. -
Intrinsic Point Defects in Ultrathin Layered 1T-Ptse2
Intrinsic Point Defects in Ultrathin Layered 1T-PtSe2 Husong Zheng1†, Yichul Choi1†, Fazel Baniasadi1,2†, Dake Hu3†, Liying Jiao3*, Kyungwha Park1*, Chenggang Tao1* 1Department of Physics, Virginia Tech, Blacksburg, Virginia 24061, USA 2Department of Materials Science and Engineering, Virginia Tech, Blacksburg, Virginia 24061, USA 3Department of Chemistry, Tsinghua University, Beijing 100084, China †These authors contributed equally to this work. *Corresponding Authors: [email protected], [email protected] and [email protected]. 1 ABSTRACT Among two-dimensional (2D) transition metal dichalcogenides (TMDs), platinum diselenide (PtSe2) stands at a unique place in the sense that it undergoes a phase transition from type-II Dirac semimetal to indirect-gap semiconductor as thickness decreases. Defects in 2D TMDs are ubiquitous and play crucial roles in understanding and tuning electronic, optical, and magnetic properties. Here we investigate intrinsic point defects in ultrathin 1T-PtSe2 layers grown on mica through the chemical vapor transport (CVT) method, using scanning tunneling microscopy and spectroscopy (STM/STS) and first-principles calculations. We observed five types of distinct defects from STM topography images and obtained the local density of states of the defects. By combining the STM results with the first-principles calculations, we identified the types and characteristics of these defects, which are Pt vacancies at the topmost and next monolayers, Se vacancies in the topmost monolayer, and Se antisites at Pt sites within the topmost monolayer. Our study shows that the Se antisite defects are the most abundant with the lowest formation energy in a Se-rich growth condition, in contrast to cases of 2D molybdenum disulfide (MoS2) family. -
Entire Research Accomplishments
250 Duffield Hall • 343 Campus Road • Ithaca NY 14853-2700 Phone: 607.255.2329 • Fax: 607.255.8601 • Email: [email protected] • Website: www.cnf.cornell.edu Cornell NanoScale Facility 2017-2018 Research Accomplishments CNF Lester B. Knight Director: Christopher Ober Director of Operations: Donald Tennant Cornell NanoScale Facility (CNF) is a member of the National Nanotechnology Coordinated Infrastructure (www.nnci.net) and is supported by the National Science Foundation under Grant No. NNCI-1542081, the New York State Office of Science, Technology and Academic Research, Cornell University, Industry, and our Users. The 2017-2018 CNF Research Accomplishments are also available on the web: http://www.cnf.cornell.edu/cnf_2018cnfra.html © 2018 2017-2018 Research Accomplishments i Cornell NanoScale Science & Technology Facility 2017-2018 Research Accomplishments Table of Contents Technical Reports by Section. ... ... ... ... ... ... ... ... ... ... ... ... ... ... ... ... ... ... ... ... .ii Directors' Introduction .................................................................... vi A Selection of 2017 Patents, Presentations & Publications ... ... ... ... ... ... ... ... ix Photography Credits ... ... ... ... ... ... ... ... ... ... ... ... ... ... ... ... ... ... ... ... ... ... ...xxvii Full Color Versions of a few Research Images.............................. xxviii-xxix Abbreviations & Their Meanings ... ... ... ... ... ... ... ... ... ... ... ... ... ... ... ... ... ... xxx Technical Reports ... ... ... ... ... ... ... .. -
Synthesis and Characterization of Transition Metal Oxide And
University of Wisconsin Milwaukee UWM Digital Commons Theses and Dissertations May 2018 Synthesis and Characterization of Transition Metal Oxide and Dichalcogenide Nanomaterials for Energy and Environmental Applications Ren Ren University of Wisconsin-Milwaukee Follow this and additional works at: https://dc.uwm.edu/etd Part of the Materials Science and Engineering Commons, and the Mechanical Engineering Commons Recommended Citation Ren, Ren, "Synthesis and Characterization of Transition Metal Oxide and Dichalcogenide Nanomaterials for Energy and Environmental Applications" (2018). Theses and Dissertations. 1909. https://dc.uwm.edu/etd/1909 This Dissertation is brought to you for free and open access by UWM Digital Commons. It has been accepted for inclusion in Theses and Dissertations by an authorized administrator of UWM Digital Commons. For more information, please contact [email protected]. SYNTHESIS AND CHARACTERIZATION OF TRANSITION METAL OXIDE AND DICHALCOGENIDE NANOMATERIALS FOR ENERGY AND ENVIRONMENTAL APPLICATIONS by Ren Ren A Dissertation Submitted in Partial Fulfillment of the Requirements for the Degree of Doctor of Philosophy in Engineering at The University of Wisconsin-Milwaukee May 2018 ABSTRACT SYNTHESIS AND CHARACTERIZATION OF TRANSITION METAL OXIDE AND DICHALCOGENIDE NANOMATERIALS FOR ENERGY AND ENVIRONMENTAL APPLICATIONS by Ren Ren The University of Wisconsin-Milwaukee, 2018 Under the Supervision of Professor Junhong Chen Transition metal oxides (TMOs) and transition metal dichalcogenides (TMDs) have gained immense interest recently for energy and environmental applications due to their exceptional structural, electronic, and optical properties. For example, titanium dioxide (TiO2) as one of the TMO photocatalysts has been widely studied due to its stability, non-toxicity, wide availability, and high efficiency. However, its wide bandgap significantly limits its use under visible light or solar light.