1 Introduction
Total Page:16
File Type:pdf, Size:1020Kb
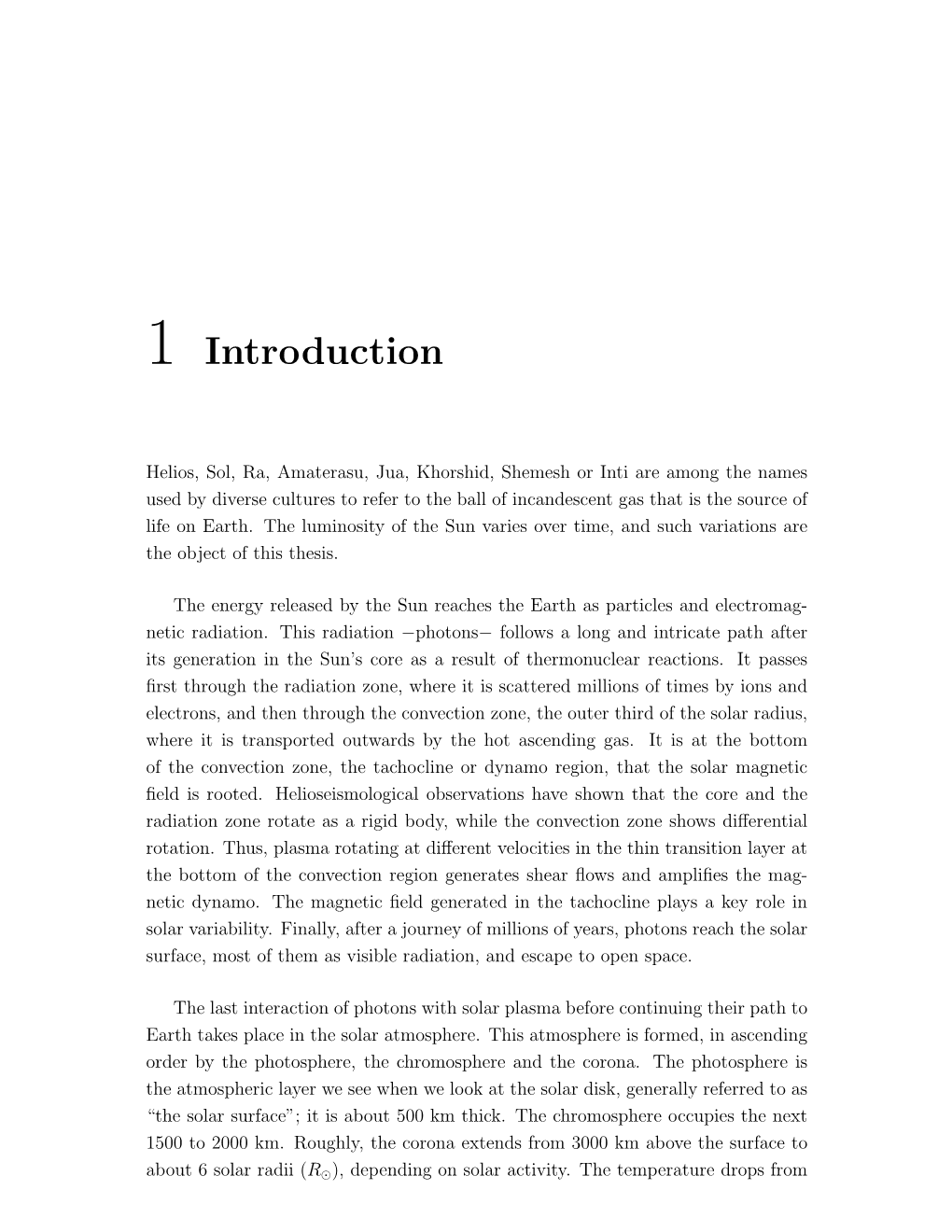
Load more
Recommended publications
-
On the Structure of Polar Faculae on the Sun
A&A 425, 321–331 (2004) Astronomy DOI: 10.1051/0004-6361:20041120 & c ESO 2004 Astrophysics On the structure of polar faculae on the Sun O. V. Okunev1,2 and F. Kneer1 1 Universitäts-Sternwarte, Geismarlandstr. 11, 37083 Göttingen, Germany e-mail: [Kneer;olok]@uni-sw.gwdg.de 2 Central Astronomical Observatory at Pulkovo, 196140 St. Petersburg, Russia Received 20 April 2004 / Accepted 25 May 2004 Abstract. Faculae on the polar caps of the Sun, in short polar faculae (PFe), are investigated. They take part in the magnetic solar cycle. Here, we study the fine structures of PFe, their magnetic fields and their dynamics on short time scales. The observations stem from several periods in 2001 and 2002. They consist of spectropolarimetric data (Stokes I and V) taken in the Fe 6301.5 and 6302.5 Å and Fe 6149.3 Å lines with the Gregory-Coudé Telescope (GCT) and the Vacuum Tower Telescope (VTT) at the Observatorio del Teide on Tenerife. At the VTT, the “Göttingen” two-dimensional Fabry-Perot spectrometer was used. It allows image reconstruction with speckle methods resulting in spatial resolution of approximately 0. 25 for broadband images and 0. 5 for magnetograms. The application of singular value decomposition yielded a polarimetric detection limit of −3 |V|≈2 × 10 Ic.WefindthatPFe,ofsizeof1 or larger, possess substantial fine structure of both brightness and magnetic fields. The brightness and the location of polar facular points change noticeably within 50 s. The facular points have strong, kilo-Gauss magnetic fields, they are unipolar with the same polarity as the global, poloidal magnetic field. -
From the Heliosphere Into the Sun Programme Book Incuding All
511th WE-Heraeus-Seminar From the Heliosphere into the Sun –SailingagainsttheWind– Programme book incuding all abstracts Physikzentrum Bad Honnef, Germany January 31 – February 3, 2012 http://www.mps.mpg.de/meetings/heliocorona/ From the Heliosphere into the Sun A meeting dedicated to the progress of our understanding of the solar wind and the corona in the light of the upcoming Solar Orbiter mission This meeting is dedicated to the processes in the solar wind and corona in the light of the upcoming Solar Orbiter mission. Over the last three decades there has been astonishing progress in our understanding of the solar corona and the inner heliosphere driven by remote-sensing and in-situ observations. This period of time has seen the first high-resolution X-ray and EUV observations of the corona and the first detailed measurements of the ion and electron velocity distribution functions in the inner heliosphere. Today we know that we have to treat the corona and the wind as one single object, which calls for a mission that is fully designed to investigate the interwoven processes all the way from the solar surface to the heliosphere. The meeting will provide a forum to review the advances over the last decades, relate them to our current understanding and to discuss future directions. We will concentrate one day on in- situ observations and related models of the inner heliosphere, and spend another day on remote sensing observations and modeling of the corona – always with an eye on the symbiotic nature of the two. On the third day we will direct our view towards the future. -
ARE DRONES a FORCE for GOOD? How Drones Are Being Used to Benefit Society
BRIEF FOOD AND DRINK CULTURE GEAR SPORTS STYLE WOMEN HOME > BANNER- HOME > MAGAZINE ARE DRONES A FORCE FOR GOOD? How drones are being used to benefit society By Matthew Priest Words: Max Mueller NEWSLETTER SUBSCRIPTION Get the lowdown on the week ahead to your inbox, together with reviews, exclusive competitions and the occasional funny list. Enter Your Email SUBSCRIBE MOST POPULAR THIS WEEK Read Shared Galleries HOW TO BULK UP LIKE A RUGBY PLAYER Drones usually feature in the news due to their controversial use as weapons of war or, more prosaically, for their use as fun but largely impractical toys. But what if the technology could be used to help the environment or save lives? This month, the UAE is again promoting the humanitarian use of drones with a $1 million competition that features the best designs from around the world. Here is a bird’s eye view of the technology and its pitfalls, in the Middle East and beyond. *** WHAT I’VE LEARNT: FLAVIO BRIATORE Fukushima Daiichi nuclear power station, April 10, 2015. Four years after the March 2011 meltdown, a stream of images is being recorded inside reactor No 1, one of three that was badly damaged after the plant was hit by a devastating tsunami. As the remote-controlled robot crawls through debris, the on-board searchlight struggles to penetrate a thick dust cloud. It also has to navigate charred concrete girders coated in solidified molten metal that now resemble warped stalactites. CESARO: 8 THINGS WE’VE At the bottom of the video screen is a digital display of the radiation exposure. -
Planning of Nuclear Power Systems
ASKO VUORINEN Planning of Nuclear Power Systems To Save the Planet Ekoenergo Oy August, 2011 The nuclear power could generate 27 % of electricity by 2050 and 34 % by 2075. Nuclear electricity generation can make the biggest change in reducing greenhouse gas emissions and it would be possible to limit the global temperature increase to 2 degrees Celsius by the year 2100. 1 Copyright © 2011 Ekoenergo Oy Lokirinne 8 A 25, 02320 Espoo, Finland Telephone (+358) 440451022 The book is available for internet orders www.optimalpowersystems.com Email (for orders and customer service enquires): [email protected] All rights reserved. No part of this publication may be reproduced, stored in retrieval system or transmitted in any form or by any means, electronic, mechanical, photocopying, scanning or otherwise, except under terms of copyright, without the permission in writing of the Publisher. Requests to the publisher should be addressed to Ekoenergo Oy, Lokirinne 8 A 25, 02320 Espoo, Finland or emailed to [email protected]. Comments to the author can be sent directly to [email protected]. Cover page: the Planet and Atoms. Created by my son Architect Teo-Tuomas Vuorinen 2 Table of Contents PREFACE ....................................................................................................................................................................... 13 .ACKNOWLEDGEMENTS .............................................................................................................................................. -
Events in the Heliosphere Fjjtuuo- If During the Present Sunspot Cycle S
A Simulation Study of Two Major "Events in the Heliosphere fJjtUUO- if During the Present Sunspot Cycle S.-I. Akasofu1, W. Fillius2, Wei Sun1*3, C. Fry1 and M. Dryer4 J-Geophysicai l Institute and Department of Space Physics and Atmospheric Sciences. University of Alaska-Fairbanks Fairbanks, Alaska 99701 ^University of California, San Diego La Jplla, California 92093 •> On leave from Institute of Geophysics * Academia Sinica . Beijing, China 4Space Environment Laboratory, NOAA Boulder, Colorado 80303 Abstract The two major disturbances in the heliosphere during the present sunspot cycle, the event of June - August, 1982 and the event of April - June, 1978, are simulated by the method developed by Hakamada and Akasofu (1982). Specifically, we attempt to simulate effects of six major flares from three active regions in June and July, 1982 and April and May, 1978. A comparison, of the results with the solar wind observations at Pioneer 12 (~ 0.8 au), • - • -• ISEE-3 (•* 1 au), Pioneer 11 (~ 7-13 au) and Pioneer 10 (~ 16-28 au) suggests that some major flares occurred behind the disk of the sun during the two periods. Our method provides qualitatively some information as to how such a series of intense solar flares can greatly disturb both the inner and outer heliospheres. A long lasting effect on cosmic rays is discussed in conjunction with the disturbed heliosphere. fNaS&-CB-1769«3) A SIHDLallCB S10DY OF THO N86-29756 MiJOB EVENTS IN TEE HELIOSPBEEE DDBING THE PRESENT SOHSP01 CYCLE (Alaska Oniv. Fairbanks.) 48 p CSCL 03B Dncla. £v 1. Introduction The months of June and July, 1982 and of April and May, 1978 were two of the* most active periods of the sun during the present solar cycle. -
The Wright Stuff
1203cent.qxd 11/13/03 2:19 PM Page 1 n a chilly North Carolina edge. It is likely that this informa- Omorning 100 years ago, two tion became the basis for the design brothers from Dayton, Ohio, at- of their early gliders. It also led them tempted a feat others considered im- to contact Octave Chanute, an possible, and their success changed American engineer who was the world. leading the way for experiments in Today we take air travel for aeronautics. granted, and rarely give a second thought to our capability to fly liter- Three problems ally anywhere in the world. But one The Wrights realized from the be- hundred years ago, the endeavors ginning that they had to solve three of the Wrights and other aeronau- problems: tical pioneers were widely viewed • Balance and control. as foolhardy. Although some of the THE WRIGHT • Wing shape and resulting lift. world’s most creative minds were • Application of power to the converging on a solution to the flight structure. problem, well-respected scientists STUFF: Of the three, they correctly recog- such as Lord Kelvin thought flight Materials in the Wright Flyer nized that balance and control were impossible. the least understood and probably In fact, Simon Newcomb, pro- The flight of the Wright Flyer was the most critical. To solve that fessor of mathematics and as- the “first in the history of the world problem, they turned to gliding ex- tronomy at Johns Hopkins Univer- periments. sity and vice-president of the in which a machine carrying a man National Academy of Sciences, had had raised itself by its own power The 1900 glider declared only 18 months before the into the air in full flight, had sailed After a few preliminary experi- successful flight at Kitty Hawk, forward without reduction of speed, ments with small kites, they built “Flight by machines heavier than air and had finally landed at a point as their first glider in 1900. -
A Forecast of Reduced Solar Activity and Its Implications for Nasa
A FORECAST OF REDUCED SOLAR ACTIVITY AND ITS IMPLICATIONS FOR NASA Kenneth Schatten and Heather Franz* a.i. solutions, Inc. ABSTRACT The “Solar Dynamo” method of solar activity forecasting is reviewed. Known generically as a “precursor” method, insofar as it uses observations which precede solar activity generation, this method now uses the Solar Dynamo Amplitude (SODA) Index to estimate future long-term solar activity. The peak amplitude of the next solar cycle (#24), is estimated at roughly 124 in terms of smoothed F10.7 Radio Flux and 74 in terms of the older, more traditional smoothed international or Zurich Sunspot number (Ri or Rz). These values are significantly smaller than the amplitudes of recent solar cycles. Levels of activity stay large for about four years near the peak in smoothed activity, which is estimated to occur near the 2012 timeflame. Confidence is added to the prediction of low activity by numerous examinations of the Sun’s weakened polar field. Direct measurements are obtained by the Mount Wilson Solar Observatory and the Wilcox Solar Observatory. Further support is obtained by examining the Sun’s polar faculae (bright features), the shape of coronal soft X-ray “holes,” and the shape of the “source surface” - a calculated coronal feature which maps the large scale structure of the Sun’s field. These features do not show the characteristics of well-formed polar coronal holes associated with typical solar minima. They show stunted polar field levels, which are thought to result in stunted levels of solar activity during solar cycle #24. The reduced levels of solar activity would have concomitant effects upon the space environment in which satellites orbit. -
Oscillations Accompanying a He I 10830 {\AA} Negative Flare in a Solar
Draft version November 9, 2018 Typeset using LATEX twocolumn style in AASTeX61 OSCILLATIONS ACCOMPANYING A HE I 10830 A˚ NEGATIVE FLARE IN A SOLAR FACULA Andrei Chelpanov1 and Nikolai Kobanov1 1Institute of Solar-Terrestrial Physics of Siberian Branch of Russian Academy of Sciences, Irkutsk, Russia ABSTRACT On September 21, 2012, we carried out spectral observations of a solar facula in the Si i 10827 A,˚ He i 10830 A,˚ and Hα spectral lines. Later, in the process of analyzing the data, we found a small-scale flare in the middle of the time series. Due to an anomalous increase in the absorption of the He i 10830 A˚ line, we identified this flare as a negative flare. The aim of this paper is to study the influence of the negative flare on the oscillation characteristics in the facular photosphere and chromosphere. We measured line-of-sight (LOS) velocity and intensity of all the three lines as well as half-width of the chromospheric lines. We also used SDO/HMI magnetic field data. The flare caused modulation of all the studied parameters. In the location of the negative flare, the amplitude of the oscillations increased four times on average. In the adjacent magnetic field local maxima, the chromospheric LOS velocity oscillations appreciably decreased during the flare. The facula region oscillated as a whole with a 5-minute period before the flare, and this synchronicity was disrupted after the flare. The flare changed the spectral composition of the line-of-sight magnetic field oscillations, causing an increase in the low-frequency oscillation power. -
Journal of Physics & Astronomy
Journal of Physics & Astronomy Review| Vol 6 Iss 3 3D Analytical Model of Steady Solar Faculae Solov'ev АА 1,2* 1Central astronomical observatory of Russian Academy of Science, S-Petersburg, Russia 2Kalmyk State University, Elista, Russia *Corresponding author: Solov'ev АА, Central astronomical observatory of Russian Academy of Science, S-Petersburg, Russia, Tel:+7 981 7170338; E-Mail: [email protected] Received: July 31, 2018; Accepted: August 3, 2018; Published: August 10, 2018 Abstract Solar facular nodes regarded as relatively stable and long-lived bright active formations with a diameter from 3 to 8 Mm and having a fine (about 1 Mm or less) magnetic filamentary structure with magnetic field strengths from 250 G to 1000 G are modeled analytically. The stationary MHD problem is solved and analytical formulae are derived that allow one to calculate the pressure, density, temperature, and Alfven Mach number in the configuration under study from the corresponding magnetic field structure. The facular node is introduced in a hydrostatic atmosphere defined by the Avrett & Loeser model and is surrounded by a weak (2G) external field corresponding to the global magnetic field intensity on the solar surface. The calculated temperature profiles of the facular node at the level of the photosphere have a characteristic shape where the temperature on the facula axis is lower than that in the surroundings but in the nearest vicinities of the axis and at the periphery of the node, the gas is 200-100 K hotter than the surroundings. Here, on the level of photosphere, the model well describes not only the central darkening of the faculae (like Wilson depression, as in sunspots), but also ring, semi-ring and segmental facular brightening observed with New Swedish 1-m Telescope at high angular resolution. -
WENDELSTEIN Solar Observatory
WENDELSTEIN Solar Observatory Daily drawings of solar features for the years 1947-1987 are available. These lovely images give a composite picture of various solar phenomena, including the solar coronal intensity, the bright Calcium plages, and the filaments and prominences, as well as sunspots. Many stations contributed data to this effort. For example, during the period April-June 1969, stations contributing Hydrogen-alpha images included Anacapri, Athens, Burbank, Catania, Freiburg, Haleakala, Kodaikanal, Sacramento Peak, Teheran, Tonantzintla, and Wendelstein. Those contributing Calcium K3 line images include Anacapri, Arcetri, Catania, Kodaikanal, Manila, Rome, and Wendelstein. Those contributing solar corona 530.3 nm data include Mt. Norikura, Pic du Midi, Sacramento Peak, and Wendelstein. North is at the top. East is on the left. A Stonyhurst disk with the correct Bo angle is used. The times of the different observations are indicated on the left side. Solar flare events are also listed, including begin and end times and position. Times that are underlined are certain. Times without underlines are uncertain. Coronal intensities are marked in numerical form every five degrees around the solar disk. On the disk’s edge, prominences appear in red. On the disk, the filaments are drawn in black, the calcium plage borders are drawn in blue, sunspots are in black, and something (?) is drawn in green. The sunspot Zurich classifications A, B, C, D, E, F, G, H, and J are indicated. To the right of the drawing are listed the positions and Zurich class of sunspots. . -
Arxiv:1707.00411V1
Solar Physics DOI: 10.1007/•••••-•••-•••-••••-• Long-term Variations in the Intensity of Plages and Networks as Observed in Kodaikanal Ca-K Digitized Data Muthu Priyal1 · Jagdev Singh2 · Ravindra B2 · Rathina S. K3 c Springer •••• Abstract In our previous article (Priyal et al., Solar Phys., 289, 127) we have discussed the details of observations and methodology adopted to analyze the Ca-K spectroheliograms obtained at Kodaikanal Observatory (KO) to study the variation of Ca-K plage areas, enhanced network (EN) and active network (AN) for the three solar cycles, namely 19, 20, and 21. Now, we have derived the areas of chromospheric features using KO Ca-K spectroheliograms to study the long term variations of solar cycles between 14 and 21. The comparison of the derived plage areas from the data obtained at KO observatory for the period 1906 – 1985 with that of MWO, NSO for the period 1965 – 2002, earlier measurements made by Tlatov, Pevtsov, and Singh (2009, Solar Phys., 255, 239) for KO data and the SIDC sunspot numbers shows a good correlation. Uniformity of the data obtained with the same instrument remaining with the same specifications provided a unique opportunity to study long term intensity variations in plages and network regions. Therefore, we have investigated the variation of intensity contrast of these features with time at a temporal resolution of 6-months assum- ing the quiet background chromosphere remains unchanged during the period of 1906 – 2005 and found that average intensity of AN, representing the changes in small scale activity over solar surface, varies with solar cycle being less during the minimum phase. -
Building Envelope Applications Utilizing Drone Technologies
Raymond Engineering – Georgia, Inc. 401104237 DRONING ON ABOUT DRONES Ray Ramos, PE July 12, 2017 Credit(s) earned on completion of this course will be reported to AIA CES for AIA members. Certificates of Completion for both AIA members and non-AIA members are available upon request. This course is registered with AIA CES for continuing professional education. As such, it does not include content that may be deemed or construed to be an approval or endorsement by the AIA of any material of construction or any method or manner of handling, using, distributing, or dealing in any material or product. __________________________________________ Questions related to specific materials, methods, and services will be addressed at the conclusion of this presentation. Copyright Materials This presentation is protected by US and International Copyright laws. Reproduction, distribution, display and use of the presentation without written permission of the speaker is prohibited. Course This course gives the audience an understanding of the history of drones and associated technology; and how the development and use of drones have evolved over time. Additionally, the course includes performing an actual drone demonstration showing how a drone currently works and how they can be used by building/property owners, architects, engineers, consultants, and/or contractors. Learning At the end of the this course, participants will be able to: • Learning Objective 1: Understanding how drones got their start and have evolved over time. • Learning Objective 2: Overview of the types of drones and uses. • Learning Objective 3: Use and operations issues and how to resolve them • Learning Objective 4: To perform a drone demonstration in order to showcase it’s capabilities.