The Extracellular Matrix As a Target for Biophysical and Molecular Magnetic Resonance Imaging
Total Page:16
File Type:pdf, Size:1020Kb
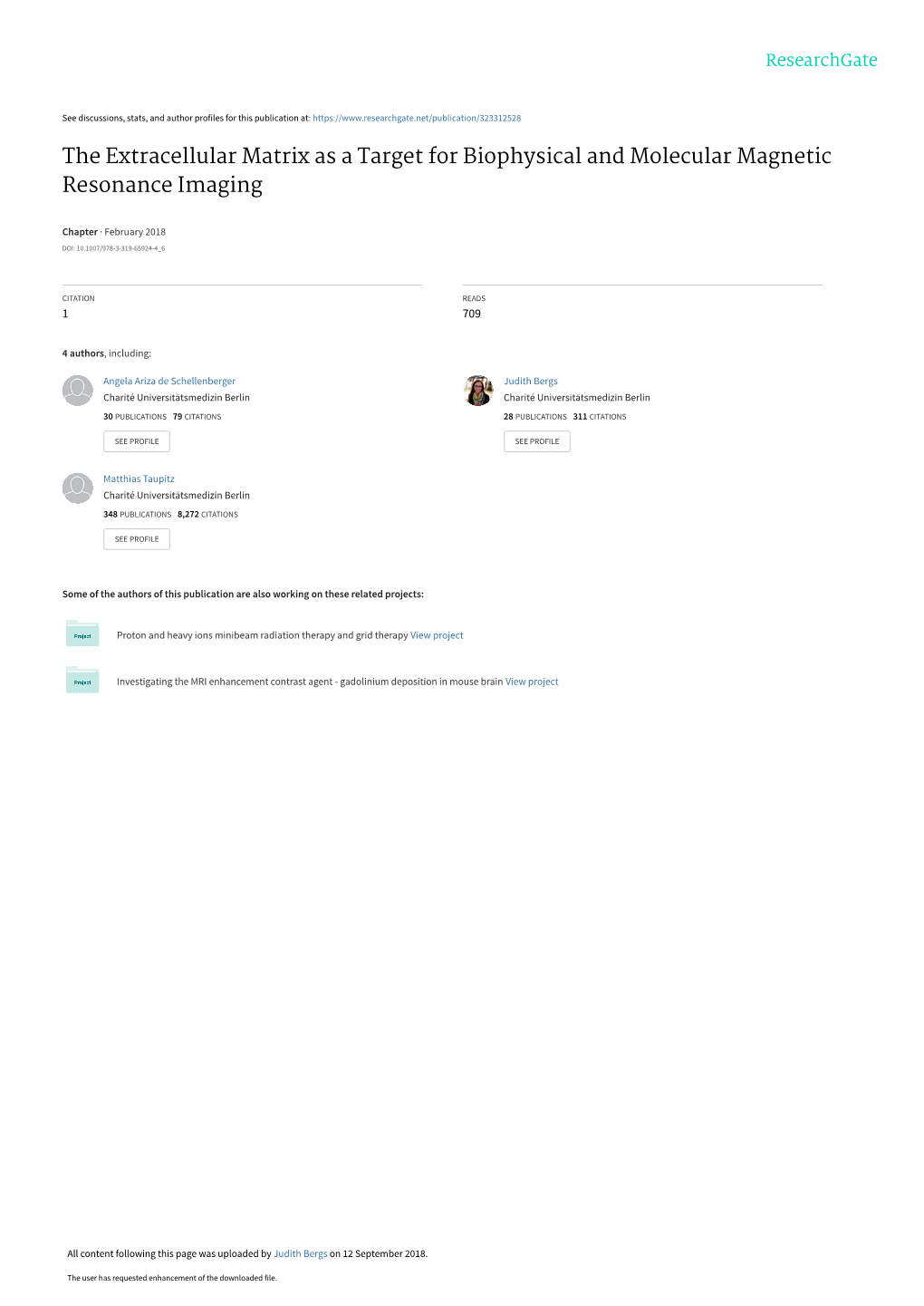
Load more
Recommended publications
-
Repair, Regeneration, and Fibrosis Gregory C
91731_ch03 12/8/06 7:33 PM Page 71 3 Repair, Regeneration, and Fibrosis Gregory C. Sephel Stephen C. Woodward The Basic Processes of Healing Regeneration Migration of Cells Stem cells Extracellular Matrix Cell Proliferation Remodeling Conditions That Modify Repair Cell Proliferation Local Factors Repair Repair Patterns Repair and Regeneration Suboptimal Wound Repair Wound Healing bservations regarding the repair of wounds (i.e., wound architecture are unaltered. Thus, wounds that do not heal may re- healing) date to physicians in ancient Egypt and battle flect excess proteinase activity, decreased matrix accumulation, Osurgeons in classic Greece. The liver’s ability to regenerate or altered matrix assembly. Conversely, fibrosis and scarring forms the basis of the Greek myth involving Prometheus. The may result from reduced proteinase activity or increased matrix clotting of blood to prevent exsanguination was recognized as accumulation. Whereas the formation of new collagen during the first necessary event in wound healing. At the time of the repair is required for increased strength of the healing site, American Civil War, the development of “laudable pus” in chronic fibrosis is a major component of diseases that involve wounds was thought to be necessary, and its emergence was not chronic injury. appreciated as a symptom of infection but considered a positive sign in the healing process. Later studies of wound infection led The Basic Processes of Healing to the discovery that inflammatory cells are primary actors in the repair process. Although scurvy (see Chapter 8) was described in Many of the basic cellular and molecular mechanisms necessary the 16th century by the British navy, it was not until the 20th for wound healing are found in other processes involving dynamic century that vitamin C (ascorbic acid) was found to be necessary tissue changes, such as development and tumor growth. -
Adenovirus-Mediated Transfer of Type IV Collagen Α5 Chain Cdna
Gene Therapy (2001) 8, 882–890 2001 Nature Publishing Group All rights reserved 0969-7128/01 $15.00 www.nature.com/gt RESEARCH ARTICLE Adenovirus-mediated transfer of type IV collagen ␣5 chain cDNA into swine kidney in vivo: deposition of the protein into the glomerular basement membrane P Heikkila¨1, A Tibell2, T Morita1, Y Chen1,GWu2, Y Sado4, Y Ninomiya5, E Pettersson3 and K Tryggvason1 1Division of Matrix Biology, Department of Medical Biochemistry and Biophysics, Departments of 2Transplantation Surgery, and 3Nephrology, Huddinge Hospital, Karolinska Institutet, Stockholm, Sweden; 4Division of Immunology, Shigei Medical Research Institute, Okayama; 5Department of Molecular Biology and Biochemistry, Okayama University Medical School, Okayama, Japan Gene therapy of Alport syndrome (hereditary nephritis) aims a FLAG epitope in the recombinant ␣5(IV) chain. The results at the transfer of a corrected type IV collagen ␣ chain gene indicate that correction of the molecular defect in Alport syn- into renal glomerular cells responsible for production of the drome is possible. Previously, we had developed an organ glomerular basement membrane (GBM). A GBM network perfusion method for effective in vivo gene transfer into composed of type IV collagen molecules is abnormal in glomerular cells. In vivo perfusion of pig kidneys with the Alport syndrome which leads progressively to kidney failure. recombinant adenovirus resulted in expression of the ␣5(IV) The most common X-linked form of the disease is caused chain in kidney glomeruli as shown by in situ hybridization by mutations in the gene for the ␣5(IV) chain, the ␣5 chain and its deposition into the GBM was shown by immunohisto- of type IV collagen. -
Premature Aggregation of Type IV Collagen and Early Lethality in Lysyl Hydroxylase 3 Null Mice
Premature aggregation of type IV collagen and early lethality in lysyl hydroxylase 3 null mice Kati Rautavuoma*†‡, Kati Takaluoma*†‡, Raija Sormunen§, Johanna Myllyharju*†, Kari I. Kivirikko*†, and Raija Soininen†¶ *Collagen Research Unit and Departments of †Medical Biochemistry and Molecular Biology and §Pathology, Biocenter Oulu, University of Oulu, 90014 Oulu, Finland Edited by Erkki Ruoslahti, The Burnham Institute, La Jolla, CA, and approved August 17, 2004 (received for review July 9, 2004) Collagens carry hydroxylysine residues that act as attachment sites LH3 is essential for the synthesis of type IV collagen during early for carbohydrate units and are important for the stability of development and, hence, for the stability of basement mem- crosslinks but have been regarded as nonessential for vertebrate branes (BMs). survival. We generated mice with targeted inactivation of the gene for one of the three lysyl hydroxylase isoenzymes, LH3. The null Materials and Methods embryos developed seemingly normally until embryonic day 8.5, Targeting of the Plod3 Gene and Generation of Mutant Mice. The but development was then retarded, with death around embryonic genomic clone used to clone the targeting construct was isolated day 9.5. Electron microscopy (EM) revealed fragmentation of base- from a 129SV mouse genomic library with human LH3 cDNA ment membranes (BMs), and immuno-EM detected type IV collagen (5) as a probe. The construct contains 1.2- and 5-kb genomic within the dilated endoplasmic reticulum and in extracellular arms and the -gal-PGK-neo cassette inserted in-frame into exon aggregates, but the typical BM staining was absent. Amorphous 1. Embryonic stem cells were targeted, and mice were generated intracellular and extracellular particles were also seen by collagen by standard techniques. -
Original Article Glycation of Matrix Proteins in the Artery Inhibits Migration of Smooth Muscle Cells from the Media to the Intima
Original Article Glycation of Matrix Proteins in the Artery Inhibits Migration of Smooth Muscle Cells from the Media to the Intima (glycation / advanced glycation end products / collagen / elastin / myocytes / atherosclerosis) A. KUZAN1, O. MICHEL1, A. GAMIAN1,2 1Department of Medical Biochemistry, Wroclaw Medical University, Wrocław, Poland 2Laboratory of Medical Microbiology, Ludwik Hirszfeld Institute of Immunology and Experimental Therapy, Polish Academy of Sciences, Wroclaw, Poland Abstract. Formation and growth of atherosclerotic Introduction plaques have serious clinical consequences. One mechanism that occurs during atherogenesis is mi- Smooth muscle cells (SMC) are predominantly lo- gration of smooth muscle cells from the middle layer cated in the middle layer of the artery, where they play a of the artery to the intima, where they proliferate crucial role: they determine the contractility of the tis- and are transformed into foam cells. This degenera- sue, hereby adjusting the blood flow through the artery. tive process is accompanied by glycation, by which In such a case, they are called contractile smooth muscle proteins are modified and change the biomechanical cells, which are characterized by the richness in actin and biochemical properties. The aim of the study filaments. They may, however, undergo phenotype was to determine whether glycation of collagen and switching and migration and become cells that reside in elastin building the walls of blood vessels alters the the intima. Then, they are called synthetic type SMC, adhesion and rate of myocyte migration. In vitro ex- are rich in rough endoplasmic reticulum and character- periments included migration assays and immuno- ized by active metabolism (Stary et al., 1992, 1994; cytochemical staining with anti α-actin, β-catenin Tukaj, 2010). -
Type IV Collagens and Basement Membrane Diseases: Cell Biology and Pathogenic Mechanisms
CHAPTER THREE Type IV Collagens and Basement Membrane Diseases: Cell Biology and Pathogenic Mechanisms Mao Mao, Marcel V. Alavi, Cassandre Labelle-Dumais and Douglas B. Gould* Departments of Ophthalmology and Anatomy, Institute for Human Genetics, UCSF School of Medicine, San Francisco, CA, USA *Corresponding author: E-mail: [email protected] Contents 1. Genomic Organization and Protein Structure of Type IV Collagens 62 1.1 Introduction and history 62 1.2 Genomic structure 64 1.3 Protein domain structure 66 1.3.1 7S domain 68 1.3.2 Triple helical domain 69 1.3.3 NC1 domain 70 2. Type IV Collagen Biosynthesis 72 2.1 Heat shock protein 47 72 2.2 Protein disulfide isomerase 73 2.3 Peptidylprolyl isomerases 74 2.4 Prolyl 4-hydroxylases 74 2.5 Prolyl 3-hydroxylases 75 2.6 Lysyl hydroxylases 76 2.7 Transport and Golgi organization 1 78 3. Type IV Collagen-Related Pathology 78 3.1 COL4A3eA6-associated pathology 78 3.1.1 Goodpasture disease 78 3.1.2 Alport syndrome 79 3.2 COL4A1/COL4A2-associated pathology 81 3.2.1 Ocular dysgenesis 81 3.2.2 Porencephaly 82 3.2.3 Small vessel disease 83 3.2.4 Cerebral cortical lamination defects 84 3.2.5 Myopathy 85 3.2.6 HANAC syndrome and nephropathy 85 4. Mechanisms for Type IV Collagen-Related Pathology 86 4.1 Overview 86 Current Topics in Membranes, Volume 76 ISSN 1063-5823 © 2015 Elsevier Inc. http://dx.doi.org/10.1016/bs.ctm.2015.09.002 All rights reserved. 61 j 62 Mao Mao et al. -
Collagens—Structure, Function, and Biosynthesis
View metadata, citation and similar papers at core.ac.uk brought to you by CORE provided by University of East Anglia digital repository Advanced Drug Delivery Reviews 55 (2003) 1531–1546 www.elsevier.com/locate/addr Collagens—structure, function, and biosynthesis K. Gelsea,E.Po¨schlb, T. Aignera,* a Cartilage Research, Department of Pathology, University of Erlangen-Nu¨rnberg, Krankenhausstr. 8-10, D-91054 Erlangen, Germany b Department of Experimental Medicine I, University of Erlangen-Nu¨rnberg, 91054 Erlangen, Germany Received 20 January 2003; accepted 26 August 2003 Abstract The extracellular matrix represents a complex alloy of variable members of diverse protein families defining structural integrity and various physiological functions. The most abundant family is the collagens with more than 20 different collagen types identified so far. Collagens are centrally involved in the formation of fibrillar and microfibrillar networks of the extracellular matrix, basement membranes as well as other structures of the extracellular matrix. This review focuses on the distribution and function of various collagen types in different tissues. It introduces their basic structural subunits and points out major steps in the biosynthesis and supramolecular processing of fibrillar collagens as prototypical members of this protein family. A final outlook indicates the importance of different collagen types not only for the understanding of collagen-related diseases, but also as a basis for the therapeutical use of members of this protein family discussed in other chapters of this issue. D 2003 Elsevier B.V. All rights reserved. Keywords: Collagen; Extracellular matrix; Fibrillogenesis; Connective tissue Contents 1. Collagens—general introduction ............................................. 1532 2. Collagens—the basic structural module......................................... -
Characterization of a Type IV Collagen Major Cell Binding Site with Affinity to the Cd
View metadata, citation and similar papers at core.ac.uk brought to you by CORE provided by PubMed Central Characterization of a Type IV Collagen Major Cell Binding Site with Affinity to the cd/ l and the a2 1 Integrins Philipp Vandenberg, Andreas Kern, Albert Ries, Louise Luckenbill-Edds, Karlheinz Mann, and Klaus Kiilm Max-Planck-Institut f/Jr Biochemie, D-8033 Martinsried, Germany Abstract. The aim of this investigation was to identify closer investigation of the binding site. The smallest, the domains of type IV collagen participating in cell fully active triple-helical fragment was (150)3-amino binding and the cell surface receptor involved. A ma- acid residues long. It contained segments of 27 and 37 jor cell binding site was found in the trimeric cyanogen residues, respectively, at the NHe and COOH terminus, bromide-derived fragment CB3, located 100 nm away which proved to be essential for cell binding. from the NH: terminus of the molecule, in which the By affinity chromatography on Sepharose-immobilized triple-helical conformation is stabilized by interchain CB3, two receptor molecules of the integrin family, otl/31 disulfide bridges. Cell attachment assays with type IV and oe2/$1, were isolated. Their subunits were identified collagen and CB3 revealed comparable cell binding ac- by sequencing the NH2 termini or by immunoblotting. tivities. Antibodies against CB3 inhibited attachment The availability of fragment CB3 will allow for a more on fragment CB3 completely and on type IV collagen in-depth study of the molecular interaction of a short, to 80%. The ability to bind cells was strictly confor- well defined triple-helical ligand with collagen recep- mation dependent. -
Structure, Function and Ageing of the Collagens of the Eye
Eye (1987) 1, 175-183 Structure, Function and Ageing of the Collagens of the Eye ALLEN 1. BAILEY Bristol I. Introduci:ion twelve genetically distinct types of collagen in The collagenous tissues of the eye have mammalian tissues. They have all been charac received considerable attention since, 'apart terised biochemically and their precise from the importance ofthe organ itself, the eye location in complex tissues has been deter is composed of several highly specialised mined by immunohistochemical techniques. tissues which possess distinct collagenous The collagens identifiedto date have been clas structures. It is, in fact, a vivid example of the sified by several criteria, length of molecule, biological diversity of collagenous tissues. This molecular weight, flexibility of the molecule, diversity has recently been shown to be due to and by ultimate supramolecular structure. the existence of a whole family of collagen mol Employing the latter classification one can ecules that are capable of aggregating in dif consider three groups: the fibrous collagens, ferent ways to produce a variety of collagenous the non-fibrouscollagens and the filamentous structures.l At the present time there are about collagens. The variations in structure of these aggregates is shown in Figure 1. FffiROUS COLLAGENS Fibrous collagens. These collagens are revealed in the electron microscope as thick TypeI,n,JIl fibreswith a characteristic axial repeat pattern of 67 nm. The diameter of the fibresvaries con siderably, from 200 nm in skin and tendon to NON - FIBROUS COLLAGENS about 25 nm in the cornea. The size distri Type IV bution within a particular tissue may be uni form, for example the fibresof the cornea vary from 25 to 30 nm, or may be highly variable as in the skin, where they can vary from 20 to 200nm. -
Immunohistochemical Detection of Keratin, Actin and Type Iv Collagen in Serial Sections of Methacarn-Fixed Breast Cancer Tissues
ACTA HISTOCHEM. CYTOCHEM. Vol. 25, Nos. 1 & 2, 1992 IMMUNOHISTOCHEMICAL DETECTION OF KERATIN, ACTIN AND TYPE IV COLLAGEN IN SERIAL SECTIONS OF METHACARN-FIXED BREAST CANCER TISSUES HIDETOSENZAKI, AIRO TSUBURA, TETSUJISHOJI, HIYOSHIOKADA, HIDEKI TAKAHASHI ANDSOTOKICHI MORII Departmentof Pathology,Kansai Medical University, Moriguchi, Osaka 570 Receivedfor publication September 30, 1991 and in revised form October 29, 1991 Correlations among the immunolocalizations of keratin, actin and type IV collagen were investigated in serial sections of methacarn-fixed, paraffin-embedded mastectomy specimens taken from infiltrating breast cancer patients. Stainings of the filaments in pretreated sections with actinase were often weaker and/or less apparent in cancer cells than in normal mammary gland cells. If at least 30% of living cancer cells were stained, the case was scored positive. Positivity for 312C8-1 specific for basal cell type keratin 14 was observed in 8 of 60 cases, while for NCL-5D3 specific for luminal cell type keratins, positivity was observed in 57 of 60 cases. Five cases positive for HHF35 specific for muscle actin were also positive for 312C8-1 and NCL-5D3. Type IV collagen in cancer foci was patchy and/or absent, but small cancer nests surrounded completely by continuous type IV collagen, termed "in situ carcinoma components" were found in 25 of 60 cases. In all the components, stainings with 312C8-1 and/or NCL5D3 were found in some cancer cells, and the former was almost always seen in a basal orientation within the components. In con- clusion, keratin 14-positive basal cells could be identified in paraffin sections, and these findings suggest that the basal cells are closely related to continuous type IV collagen and a progressive loss of the cells may promote metastasis through fragmented type IV collagen. -
Endothelial Basement Membrane Components and Their Products, Matrikines: Active Drivers of Pulmonary Hypertension?
cells Review Endothelial Basement Membrane Components and Their Products, Matrikines: Active Drivers of Pulmonary Hypertension? Ayse Ceren Mutgan 1, Katharina Jandl 2,3 and Grazyna Kwapiszewska 1,2,* 1 Otto Loewi Research Center, Division of Physiology, Medical University of Graz, 8010 Graz, Austria; [email protected] 2 Ludwig Boltzmann Institute for Lung Vascular Research, 8010 Graz, Austria; [email protected] 3 Otto Loewi Research Center, Division of Pharmacology, Medical University of Graz, 8010 Graz, Austria * Correspondence: [email protected] Received: 31 July 2020; Accepted: 29 August 2020; Published: 3 September 2020 Abstract: Pulmonary arterial hypertension (PAH) is a vascular disease that is characterized by elevated pulmonary arterial pressure (PAP) due to progressive vascular remodeling. Extracellular matrix (ECM) deposition in pulmonary arteries (PA) is one of the key features of vascular remodeling. Emerging evidence indicates that the basement membrane (BM), a specialized cluster of ECM proteins underlying the endothelium, may be actively involved in the progression of vascular remodeling. The BM and its steady turnover are pivotal for maintaining appropriate vascular functions. However, the pathologically elevated turnover of BM components leads to an increased release of biologically active short fragments, which are called matrikines. Both BM components and their matrikines can interfere with pivotal biological processes, such as survival, proliferation, adhesion, and migration and thus may actively contribute to endothelial dysfunction. Therefore, in this review, we summarize the emerging role of the BM and its matrikines on the vascular endothelium and further discuss its implications on lung vascular remodeling in pulmonary hypertension. Keywords: IPAH; vascular remodeling; basement membrane; laminin; type IV collagen; matrikines; endostatin; BMPRII; barrier function; apoptosis 1. -
Mechanics and Structural Stability of the Collagen Triple Helix
Mechanics and Structural Stability of the Collagen Triple Helix Michael W.H. Kirkness,1 Kathrin Lehmann2 and Nancy R. Forde1,2 1Department of Molecular Biology and Biochemistry and 2Department of Physics, Simon Fraser University. Burnaby, BC, V5A 1S6 Canada [email protected] Abstract The primary building block of the body is collagen, which is found in the extracellular matrix and in many stress-bearing tissues such as tendon and cartilage. It provides elasticity and support to cells and tissues while influencing biological pathways including cell signaling, motility and differentiation. Collagen’s unique triple helical structure is thought to impart mechanical stability. However, detailed experimental studies on its molecular mechanics have been only recently emerging. Here, we review the treatment of the triple helix as a homogeneous flexible rod, including bend (standard worm-like chain model), twist, and stretch deformations, and the assumption of backbone linearity. Additionally, we discuss protein- specific properties of the triple helix including sequence dependence, and relate single-molecule mechanics to collagen’s physiological context. Introduction Collagen is the most abundant protein in the human body, accounting for more than 30% of the total protein.1,2 Collagens are defined by their unique right-handed triple helical structure, comprised of three left-handed polyproline-like helices, each with a (Gly-X-Y) repeating sequence where X and Y are often proline and hydroxyproline.2 In the body, collagen forms ordered, hierarchical -
Distribution of Myoepithelial Cells and Basement Membrane Proteins in the Normal Breast and in Benign and Malignant Breast Diseases
[CANCER RESEARCH 42, 4763-4770, November 1982] 0008-5472/82/0042-0000$02.00 Distribution of Myoepithelial Cells and Basement Membrane Proteins in the Normal Breast and in Benign and Malignant Breast Diseases Barry A. Gusterson,1 Michael J. Warburton, Diana Mitchell, Morag Ellison, A. Munro Neville, and Philip S. Rudland Ludwig Institute for Cancer Research (London Branch) [B. A. G., M. J. W., M. E., A, M. N., P. S. R.I and Department of Histopathology ¡D.M.], Royal Marsden Hospital, Sutton, Surrey, SM2 5PX United Kingdom ABSTRACT tins occur in epithelial cells, myosin myofilaments occur in smooth muscle cells, fibronectin is produced by stromal cells, An immunocytochemical method for fixed and paraffin- and laminin and type IV collagen are produced by endothelial embedded human breast biopsies is reported for the detection cells. This paper describes the demonstration of keratin, of myoepithelial and epithelial cells using antibodies to myosin myosin, laminin, and type IV collagen using immunocytochem and keratin, respectively, and of basement membranes using ical localization techniques with specific antibodies in fixed and antibodies to laminin and type IV collagen. Using these paraffin-embedded specimens from a range of normal, benign, markers, myoepithelial cells can be clearly distinguished in the and malignant breast biopsies. This technique yields a superior normal breast and in the benign breast diseases sclerosing cytology to that obtained previously using immunofluorescent adenosis, epitheliosis, and fibroadenoma. In sclerosing aden- localization of single antibodies on frozen breast sections and osis, myoepithelial cells form a major cellular component. A delineates the myoepithelial cell in benign breast diseases from stromally derived spindle cell is identified which stains with other reactive stromal cells, e.g., myofibroblasts.