The Crustal D Ichotom Y on Mars 1991 1
Total Page:16
File Type:pdf, Size:1020Kb
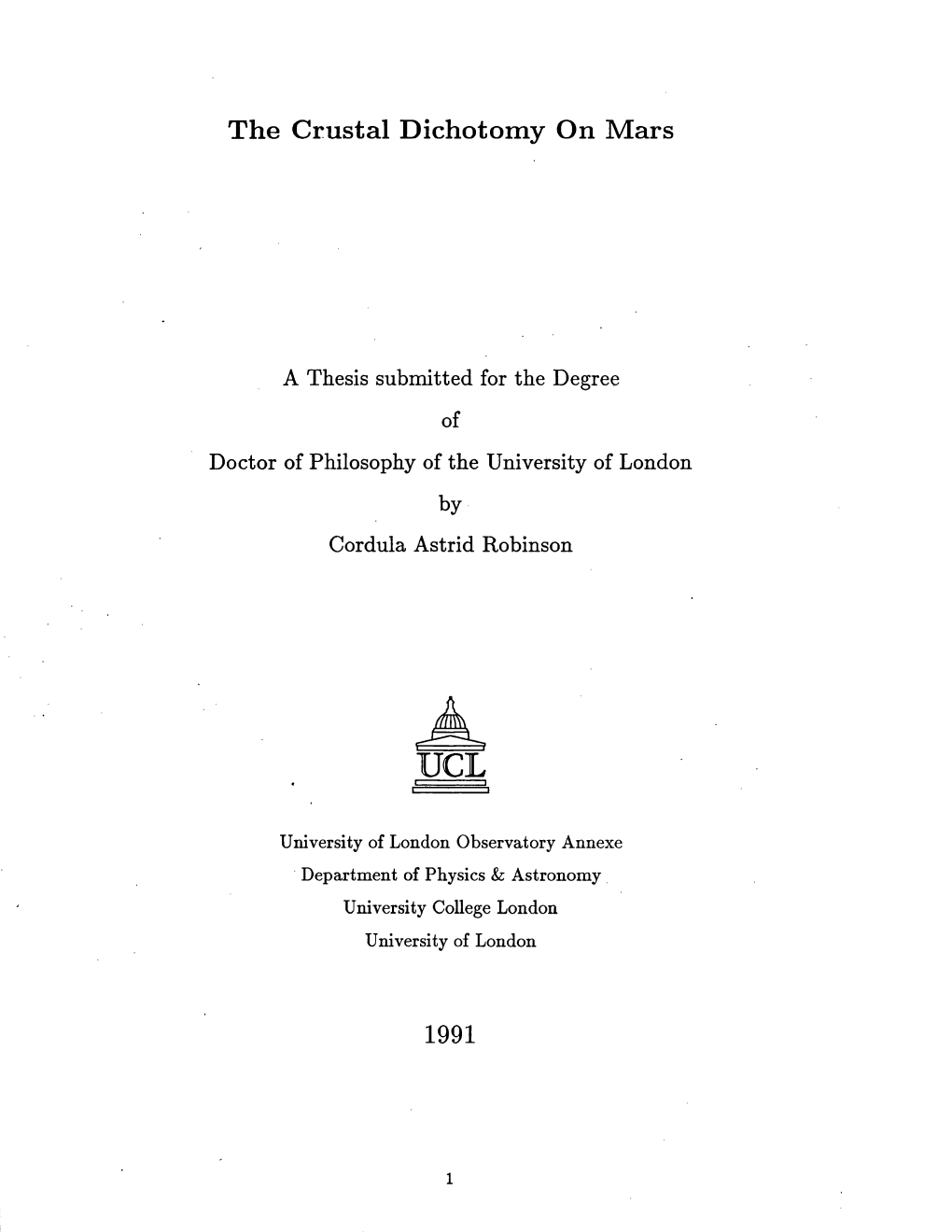
Load more
Recommended publications
-
Thermal Studies of Martian Channels and Valleys Using Termoskan Data
JOURNAL OF GEOPHYSICAL RESEARCH, VOL. 99, NO. El, PAGES 1983-1996, JANUARY 25, 1994 Thermal studiesof Martian channelsand valleys using Termoskan data BruceH. Betts andBruce C. Murray Divisionof Geologicaland PlanetarySciences, California Institute of Technology,Pasadena The Tennoskaninstrument on boardthe Phobos '88 spacecraftacquired the highestspatial resolution thermal infraredemission data ever obtained for Mars. Included in thethermal images are 2 km/pixel,midday observations of severalmajor channel and valley systems including significant portions of Shalbatana,Ravi, A1-Qahira,and Ma'adimValles, the channelconnecting Vailes Marineris with HydraotesChaos, and channelmaterial in Eos Chasma.Tennoskan also observed small portions of thesouthern beginnings of Simud,Tiu, andAres Vailes and somechannel material in GangisChasma. Simultaneousbroadband visible reflectance data were obtainedfor all but Ma'adimVallis. We find thatmost of the channelsand valleys have higher thermal inertias than their surroundings,consistent with previousthermal studies. We show for the first time that the thermal inertia boundariesclosely match flat channelfloor boundaries.Also, butteswithin channelshave inertiassimilar to the plainssurrounding the channels,suggesting the buttesare remnants of a contiguousplains surface. Lower bounds ontypical channel thermal inertias range from 8.4 to 12.5(10 -3 cal cm-2 s-1/2 K-I) (352to 523 in SI unitsof J m-2 s-l/2K-l). Lowerbounds on inertia differences with the surrounding heavily cratered plains range from 1.1 to 3.5 (46 to 147 sr). Atmosphericand geometriceffects are not sufficientto causethe observedchannel inertia enhancements.We favornonaeolian explanations of the overall channel inertia enhancements based primarily upon the channelfloors' thermal homogeneity and the strongcorrelation of thermalboundaries with floor boundaries. However,localized, dark regions within some channels are likely aeolian in natureas reported previously. -
Prime Candidate Sites for the Astrobiological Exploration of Mars According to Its Hydrogeological Evolution
PRIME CANDIDATE SITES FOR THE ASTROBIOLOGICAL EXPLORATION OF MARS ACCORDING TO ITS HYDROGEOLOGICAL EVOLUTION. Esther R. Uceda (1), Alberto G. Fairén (2), Javier Ruiz (3), James M. Dohm (4), Tayfun Öner (5), Dirk Schulze-Makuch (6), Miguel A. de Pablo (7), Jens Örmo (8), and Victor R. Baker (4,9). (1) Seminar on Planetary Sciences, Universidad Complutense de Madrid, (2) Centro de Biología Molecular, CSIC-Universidad Autónoma de Madrid, (3) Departamento de Geodinámica, Universidad Complutense de Madrid, (4) Department of Hydrology and Water Resources, University of Arizona, (5) TURKCELL Iletisim Hizmetleri A.S., Mesrutiyet Cad. No. 153, Tepebasi, Istanbul, (6) Department of Geological Sciences, University of Texas at El Paso, (7) Área de Geología, ESCET, Universidad Rey Juan Carlos, Madrid, (8) Centro de Astrobiología, CSIC-INTA, Madrid, (9) Lunar and Planetary Laboratory, University of Arizona ([email protected]) Different-sized bodies of water have been proposed to have occurred episodically in the lowlands of Mars throughout the planet’s history [1], largely related to major stages of development of Tharsis [1], [2]. These water bodies range from large oceans in the Noachian-Early Hesperian, to a minor sea in the Late Hesperian, and reduced lakes during the Amazonian. Assuming that the search for life is directly linked to the search for water, the possible biological history of Mars must have been largely influenced by the endogenetically-driven hydrogeological cycles. In consequence, terrestrial bi- ological and environmental analogues can now be placed in context with the model proposed, so contributing to draw a general approach for the history of life on Mars. -
Atypical Landslides in the Nilosyrtis Region of Mars Anthony Guimpier, Susan Conway, Nicolas Mangold
Atypical landslides in the Nilosyrtis region of Mars Anthony Guimpier, Susan Conway, Nicolas Mangold To cite this version: Anthony Guimpier, Susan Conway, Nicolas Mangold. Atypical landslides in the Nilosyrtis region of Mars. 14th Europlanet Science Congress, Oct 2020, held online, Unknown Region. 10.5194/epsc2020- 746. hal-03091587 HAL Id: hal-03091587 https://hal.archives-ouvertes.fr/hal-03091587 Submitted on 7 Jan 2021 HAL is a multi-disciplinary open access L’archive ouverte pluridisciplinaire HAL, est archive for the deposit and dissemination of sci- destinée au dépôt et à la diffusion de documents entific research documents, whether they are pub- scientifiques de niveau recherche, publiés ou non, lished or not. The documents may come from émanant des établissements d’enseignement et de teaching and research institutions in France or recherche français ou étrangers, des laboratoires abroad, or from public or private research centers. publics ou privés. EPSC Abstracts Vol. 14, EPSC2020-746, 2020, updated on 07 Jan 2021 https://doi.org/10.5194/epsc2020-746 Europlanet Science Congress 2020 © Author(s) 2021. This work is distributed under the Creative Commons Attribution 4.0 License. Atypical landslides in the Nilosyrtis region of Mars Anthony Guimpier, Susan Conway, and Nicolas Mangold Laboratoire de Planétologie et Géodynamique CNRS UMR6112, University of Nantes, France ([email protected]) Introduction:This study focuses on four unusual landslides located inside a 25 km diameter impact crater located in Nilosyrtis Mensae (Fig.1). Our initial study revealed that one of these landslides bears close similarity to a mudslide on Earth [1]. We performed a detailed morphological analysis and a comparison with terrestrial analogues to better understand their formation mechanism. -
Evidence for Volcanism in and Near the Chaotic Terrains East of Valles Marineris, Mars
43rd Lunar and Planetary Science Conference (2012) 1057.pdf EVIDENCE FOR VOLCANISM IN AND NEAR THE CHAOTIC TERRAINS EAST OF VALLES MARINERIS, MARS. Tanya N. Harrison, Malin Space Science Systems ([email protected]; P.O. Box 910148, San Diego, CA 92191). Introduction: Martian chaotic terrain was first de- ple chaotic regions are visible in CTX images (Figs. scribed by [1] from Mariner 6 and 7 data as a “rough, 1,2). These fractures have widened since the formation irregular complex of short ridges, knobs, and irregular- of the flows. The flows overtop and/or bank up upon ly shaped troughs and depressions,” attributing this pre-existing topography such as crater ejecta blankets morphology to subsidence and suggesting volcanism (Fig. 2c). Flows are also observed originating from as a possible cause. McCauley et al. [2], who were the fractures within some craters in the vicinity of the cha- first to note the presence of large outflow channels that os regions. Potential lava flows are observed on a por- appeared to originate from the chaotic terrains in Mar- tion of the floor as Hydaspis Chaos, possibly associat- iner 9 data, proposed localized geothermal melting ed with fissures on the chaos floor. As in Hydraotes, followed by catastrophic release as the formation these flows bank up against blocks on the chaos floor, mechanism of chaotic terrain. Variants of this model implying that if the flows are volcanic in origin, the have subsequently been detailed by a number of au- volcanism occurred after the formation of Hydaspis thors [e.g. 3,4,5]. Meresse et al. -
Phobos, Deimos: Formation and Evolution Alex Soumbatov-Gur
Phobos, Deimos: Formation and Evolution Alex Soumbatov-Gur To cite this version: Alex Soumbatov-Gur. Phobos, Deimos: Formation and Evolution. [Research Report] Karpov institute of physical chemistry. 2019. hal-02147461 HAL Id: hal-02147461 https://hal.archives-ouvertes.fr/hal-02147461 Submitted on 4 Jun 2019 HAL is a multi-disciplinary open access L’archive ouverte pluridisciplinaire HAL, est archive for the deposit and dissemination of sci- destinée au dépôt et à la diffusion de documents entific research documents, whether they are pub- scientifiques de niveau recherche, publiés ou non, lished or not. The documents may come from émanant des établissements d’enseignement et de teaching and research institutions in France or recherche français ou étrangers, des laboratoires abroad, or from public or private research centers. publics ou privés. Phobos, Deimos: Formation and Evolution Alex Soumbatov-Gur The moons are confirmed to be ejected parts of Mars’ crust. After explosive throwing out as cone-like rocks they plastically evolved with density decays and materials transformations. Their expansion evolutions were accompanied by global ruptures and small scale rock ejections with concurrent crater formations. The scenario reconciles orbital and physical parameters of the moons. It coherently explains dozens of their properties including spectra, appearances, size differences, crater locations, fracture symmetries, orbits, evolution trends, geologic activity, Phobos’ grooves, mechanism of their origin, etc. The ejective approach is also discussed in the context of observational data on near-Earth asteroids, main belt asteroids Steins, Vesta, and Mars. The approach incorporates known fission mechanism of formation of miniature asteroids, logically accounts for its outliers, and naturally explains formations of small celestial bodies of various sizes. -
Magazine Nº 10
Gdańsk · Sopot · Gdynia SVENSK UTGÅVA MAGAZINE SUMMER 2019 Nº 10 INTIMATE AND LUXURIOUS APARTMENTS IN SOPOT HOTEL ,,MY STORY SOPOT combines high comfort with an APARTMENTS’’ is located in an unique atmosphere of the place unique place, near the Sopot Pier, It is worth to start your day the park and the sandy beach. with our healthy and delicious Our Hotel is perfect for holidays breakfasts, always prepared from with children, a romantic weekend the highest quality products. for two or a business trip. Evenings in intimate bar with Lovers of a healthy lifestyle will good book and favourite drink in also find something for themselves your hand will be the most relax- in the park next to My Story Sopot ing ending of your day. Apartments- the ideal place for My Story Sopot Apartments morning or evening gymnastic. impresses with carefully selected For our guests there are scent and natural cosmetics with available 17 intimate suites pearls, gold, algae and valuable decorated in a subtly elegant natural oils. style with the use of the highest In addition, you can charge an quality materials. Each apartment electric vehicle in our parking lot. PÅ SVENSKA MY STORY SOPOT gans. Samtliga är byggda med APARTMENTS ligger på en fan- material av högsta kvalite som tastisk plats nära en grönskande kombinerar hög komfort med en park, en vacker sandstrand men härlig atmosfär. även den berömda träpiren. Starta dagen med en hälsosam Vårt hotell är ett perfekt bo- och nyttig frukost med bara frä- ende för en semester med bar- scha och näringsrika ingredienser nen, en romantisk weekend eller och avsluta den i vår mysigt in- varför inte en affärsresa? redda bar med ett glas i din ena Alla med en hälsosam livsstil hand och en bok i den andra. -
Review of the MEPAG Report on Mars Special Regions
THE NATIONAL ACADEMIES PRESS This PDF is available at http://nap.edu/21816 SHARE Review of the MEPAG Report on Mars Special Regions DETAILS 80 pages | 8.5 x 11 | PAPERBACK ISBN 978-0-309-37904-5 | DOI 10.17226/21816 CONTRIBUTORS GET THIS BOOK Committee to Review the MEPAG Report on Mars Special Regions; Space Studies Board; Division on Engineering and Physical Sciences; National Academies of Sciences, Engineering, and Medicine; European Space Sciences Committee; FIND RELATED TITLES European Science Foundation Visit the National Academies Press at NAP.edu and login or register to get: – Access to free PDF downloads of thousands of scientific reports – 10% off the price of print titles – Email or social media notifications of new titles related to your interests – Special offers and discounts Distribution, posting, or copying of this PDF is strictly prohibited without written permission of the National Academies Press. (Request Permission) Unless otherwise indicated, all materials in this PDF are copyrighted by the National Academy of Sciences. Copyright © National Academy of Sciences. All rights reserved. Review of the MEPAG Report on Mars Special Regions Committee to Review the MEPAG Report on Mars Special Regions Space Studies Board Division on Engineering and Physical Sciences European Space Sciences Committee European Science Foundation Strasbourg, France Copyright National Academy of Sciences. All rights reserved. Review of the MEPAG Report on Mars Special Regions THE NATIONAL ACADEMIES PRESS 500 Fifth Street, NW Washington, DC 20001 This study is based on work supported by the Contract NNH11CD57B between the National Academy of Sciences and the National Aeronautics and Space Administration and work supported by the Contract RFP/IPL-PTM/PA/fg/306.2014 between the European Science Foundation and the European Space Agency. -
North Polar Region of Mars: Advances in Stratigraphy, Structure, and Erosional Modification
Icarus 196 (2008) 318–358 www.elsevier.com/locate/icarus North polar region of Mars: Advances in stratigraphy, structure, and erosional modification Kenneth L. Tanaka a,∗, J. Alexis P. Rodriguez b, James A. Skinner Jr. a,MaryC.Bourkeb, Corey M. Fortezzo a,c, Kenneth E. Herkenhoff a, Eric J. Kolb d, Chris H. Okubo e a US Geological Survey, Flagstaff, AZ 86001, USA b Planetary Science Institute, Tucson, AZ 85719, USA c Northern Arizona University, Flagstaff, AZ 86011, USA d Google, Inc., Mountain View, CA 94043, USA e Lunar and Planetary Laboratory, University of Arizona, Tucson, AZ 85721, USA Received 5 June 2007; revised 24 January 2008 Available online 29 February 2008 Abstract We have remapped the geology of the north polar plateau on Mars, Planum Boreum, and the surrounding plains of Vastitas Borealis using altimetry and image data along with thematic maps resulting from observations made by the Mars Global Surveyor, Mars Odyssey, Mars Express, and Mars Reconnaissance Orbiter spacecraft. New and revised geographic and geologic terminologies assist with effectively discussing the various features of this region. We identify 7 geologic units making up Planum Boreum and at least 3 for the circumpolar plains, which collectively span the entire Amazonian Period. The Planum Boreum units resolve at least 6 distinct depositional and 5 erosional episodes. The first major stage of activity includes the Early Amazonian (∼3 to 1 Ga) deposition (and subsequent erosion) of the thick (locally exceeding 1000 m) and evenly- layered Rupes Tenuis unit (ABrt), which ultimately formed approximately half of the base of Planum Boreum. As previously suggested, this unit may be sourced by materials derived from the nearby Scandia region, and we interpret that it may correlate with the deposits that regionally underlie pedestal craters in the surrounding lowland plains. -
Amazonian Northern Mid-Latitude Glaciation on Mars: a Proposed Climate Scenario Jean-Baptiste Madeleine, François Forget, James W
Amazonian Northern Mid-Latitude Glaciation on Mars: A Proposed Climate Scenario Jean-Baptiste Madeleine, François Forget, James W. Head, Benjamin Levrard, Franck Montmessin, Ehouarn Millour To cite this version: Jean-Baptiste Madeleine, François Forget, James W. Head, Benjamin Levrard, Franck Montmessin, et al.. Amazonian Northern Mid-Latitude Glaciation on Mars: A Proposed Climate Scenario. Icarus, Elsevier, 2009, 203 (2), pp.390-405. 10.1016/j.icarus.2009.04.037. hal-00399202 HAL Id: hal-00399202 https://hal.archives-ouvertes.fr/hal-00399202 Submitted on 11 Apr 2016 HAL is a multi-disciplinary open access L’archive ouverte pluridisciplinaire HAL, est archive for the deposit and dissemination of sci- destinée au dépôt et à la diffusion de documents entific research documents, whether they are pub- scientifiques de niveau recherche, publiés ou non, lished or not. The documents may come from émanant des établissements d’enseignement et de teaching and research institutions in France or recherche français ou étrangers, des laboratoires abroad, or from public or private research centers. publics ou privés. 1 Amazonian Northern Mid-Latitude 2 Glaciation on Mars: 3 A Proposed Climate Scenario a a b c 4 J.-B. Madeleine , F. Forget , James W. Head , B. Levrard , d a 5 F. Montmessin , and E. Millour a 6 Laboratoire de M´et´eorologie Dynamique, CNRS/UPMC/IPSL, 4 place Jussieu, 7 BP99, 75252, Paris Cedex 05, France b 8 Department of Geological Sciences, Brown University, Providence, RI 02912, 9 USA c 10 Astronomie et Syst`emes Dynamiques, IMCCE-CNRS UMR 8028, 77 Avenue 11 Denfert-Rochereau, 75014 Paris, France d 12 Service d’A´eronomie, CNRS/UVSQ/IPSL, R´eduit de Verri`eres, Route des 13 Gatines, 91371 Verri`eres-le-Buisson Cedex, France 14 Pages: 43 15 Tables: 1 16 Figures: 13 Email address: [email protected] (J.-B. -
The 1997 Mars Pathfinder Spacecraft Landing Site: Spillover Deposits
www.nature.com/scientificreports OPEN The 1997 Mars Pathfnder Spacecraft Landing Site: Spillover Deposits from an Early Mars Inland Sea Received: 13 June 2018 J. A. P. Rodriguez1, V. R. Baker2, T. Liu 2, M. Zarroca3, B. Travis1, T. Hui2, G. Komatsu 4, Accepted: 22 January 2019 D. C. Berman1, R. Linares3, M. V. Sykes1, M. E. Banks1,5 & J. S. Kargel1 Published: xx xx xxxx The Martian outfow channels comprise some of the largest known channels in the Solar System. Remote-sensing investigations indicate that cataclysmic foods likely excavated the channels ~3.4 Ga. Previous studies show that, in the southern circum-Chryse region, their fooding pathways include hundreds of kilometers of channel foors with upward gradients. However, the impact of the reversed channel-foor topography on the cataclysmic foods remains uncertain. Here, we show that these channel foors occur within a vast basin, which separates the downstream reaches of numerous outfow channels from the northern plains. Consequently, foods propagating through these channels must have ponded, producing an inland sea, before reaching the northern plains as enormous spillover discharges. The resulting paleohydrological reconstruction reinterprets the 1997 Pathfnder landing site as part of a marine spillway, which connected the inland sea to a hypothesized northern plains ocean. Our food simulation shows that the presence of the sea would have permitted the propagation of low-depth foods beyond the areas of reversed channel-foor topography. These results explain the formation at the landing site of possible fuvial features indicative of fow depths at least an order of magnitude lower than those apparent from the analyses of orbital remote-sensing observations. -
Workshop on the Martiannorthern Plains: Sedimentological,Periglacial, and Paleoclimaticevolution
NASA-CR-194831 19940015909 WORKSHOP ON THE MARTIANNORTHERN PLAINS: SEDIMENTOLOGICAL,PERIGLACIAL, AND PALEOCLIMATICEVOLUTION MSATT ..V",,2' :o_ MarsSurfaceandAtmosphereThroughTime Lunar and PlanetaryInstitute 3600 Bay AreaBoulevard Houston TX 77058-1113 ' _ LPI/TR--93-04Technical, Part 1 Report Number 93-04, Part 1 L • DISPLAY06/6/2 94N20382"£ ISSUE5 PAGE2088 CATEGORY91 RPT£:NASA-CR-194831NAS 1.26:194831LPI-TR-93-O4-PT-ICNT£:NASW-4574 93/00/00 29 PAGES UNCLASSIFIEDDOCUMENT UTTL:Workshopon the MartianNorthernPlains:Sedimentological,Periglacial, and PaleoclimaticEvolution TLSP:AbstractsOnly AUTH:A/KARGEL,JEFFREYS.; B/MOORE,JEFFREY; C/PARKER,TIMOTHY PAA: A/(GeologicalSurvey,Flagstaff,AZ.); B/(NationalAeronauticsand Space Administration.GoddardSpaceFlightCenter,Greenbelt,MD.); C/(Jet PropulsionLab.,CaliforniaInst.of Tech.,Pasadena.) PAT:A/ed.; B/ed.; C/ed. CORP:Lunarand PlanetaryInst.,Houston,TX. SAP: Avail:CASIHC A03/MFAOI CIO: UNITEDSTATES Workshopheld in Fairbanks,AK, 12-14Aug.1993;sponsored by MSATTStudyGroupandAlaskaUniv. MAJS:/*GLACIERS/_MARSSURFACE/*PLAINS/*PLANETARYGEOLOGY/*SEDIMENTS MINS:/ HYDROLOGICALCYCLE/ICE/MARS CRATERS/MORPHOLOGY/STRATIGRAPHY ANN: Papersthathavebeen acceptedforpresentationat the Workshopon the MartianNorthernPlains:Sedimentological,Periglacial,and Paleoclimatic Evolution,on 12-14Aug. 1993in Fairbanks,Alaskaare included.Topics coveredinclude:hydrologicalconsequencesof pondedwateron Mars; morpho!ogical and morphometric studies of impact cratersin the Northern Plainsof Mars; a wet-geology and cold-climateMarsmodel:punctuation -
March 21–25, 2016
FORTY-SEVENTH LUNAR AND PLANETARY SCIENCE CONFERENCE PROGRAM OF TECHNICAL SESSIONS MARCH 21–25, 2016 The Woodlands Waterway Marriott Hotel and Convention Center The Woodlands, Texas INSTITUTIONAL SUPPORT Universities Space Research Association Lunar and Planetary Institute National Aeronautics and Space Administration CONFERENCE CO-CHAIRS Stephen Mackwell, Lunar and Planetary Institute Eileen Stansbery, NASA Johnson Space Center PROGRAM COMMITTEE CHAIRS David Draper, NASA Johnson Space Center Walter Kiefer, Lunar and Planetary Institute PROGRAM COMMITTEE P. Doug Archer, NASA Johnson Space Center Nicolas LeCorvec, Lunar and Planetary Institute Katherine Bermingham, University of Maryland Yo Matsubara, Smithsonian Institute Janice Bishop, SETI and NASA Ames Research Center Francis McCubbin, NASA Johnson Space Center Jeremy Boyce, University of California, Los Angeles Andrew Needham, Carnegie Institution of Washington Lisa Danielson, NASA Johnson Space Center Lan-Anh Nguyen, NASA Johnson Space Center Deepak Dhingra, University of Idaho Paul Niles, NASA Johnson Space Center Stephen Elardo, Carnegie Institution of Washington Dorothy Oehler, NASA Johnson Space Center Marc Fries, NASA Johnson Space Center D. Alex Patthoff, Jet Propulsion Laboratory Cyrena Goodrich, Lunar and Planetary Institute Elizabeth Rampe, Aerodyne Industries, Jacobs JETS at John Gruener, NASA Johnson Space Center NASA Johnson Space Center Justin Hagerty, U.S. Geological Survey Carol Raymond, Jet Propulsion Laboratory Lindsay Hays, Jet Propulsion Laboratory Paul Schenk,