3D Chromatin Organization Changes Modulate Adipogenesis and 2 Osteogenesis
Total Page:16
File Type:pdf, Size:1020Kb
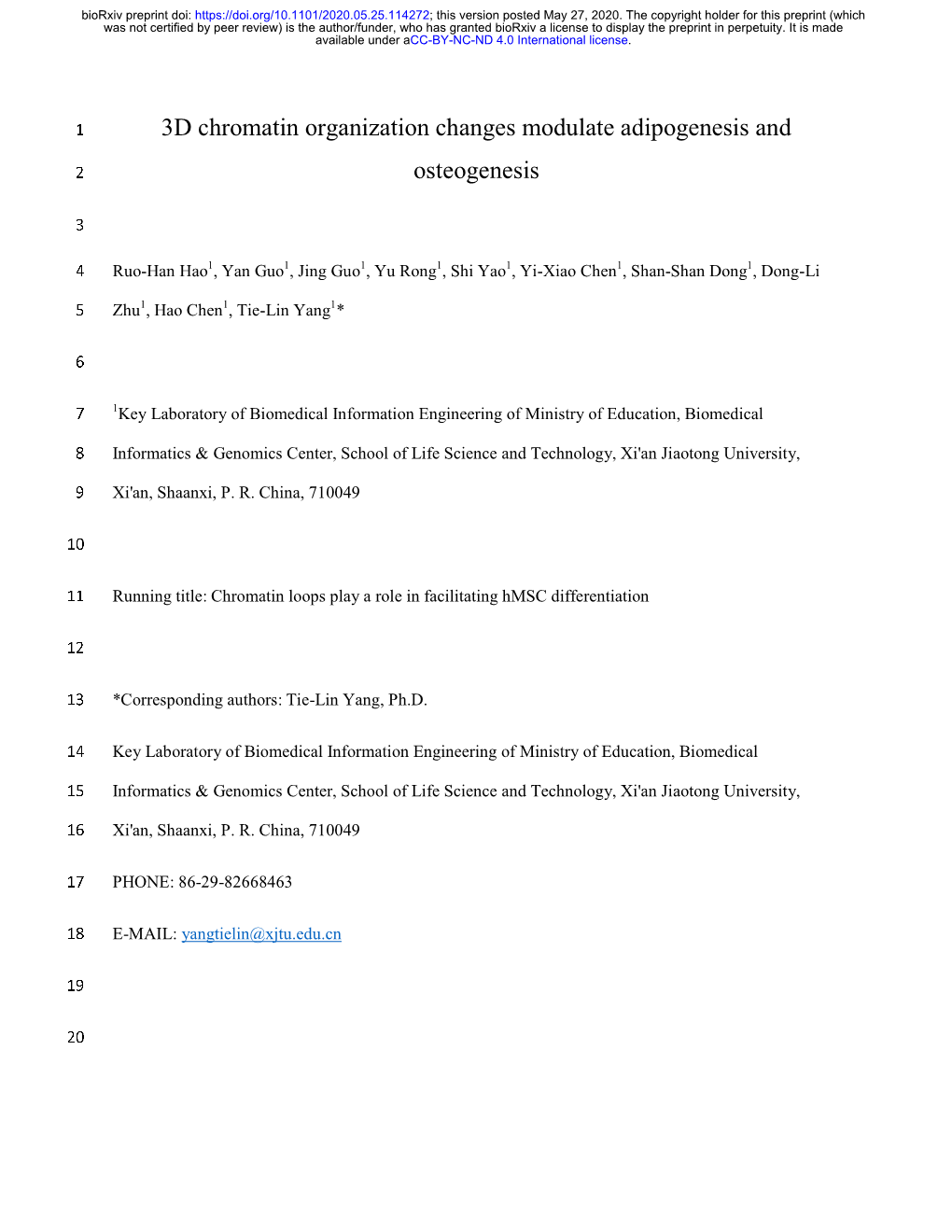
Load more
Recommended publications
-
HEXIM1 (D5Y5K) Rabbit
HEXIM1 (D5Y5K) Rabbit mAb Store at -20°C 3 n 100 µl Orders n 877-616-CELL (2355) (10 western blots) [email protected] Support n 877-678-TECH (8324) [email protected] Web n www.cellsignal.com rev. 01/05/15 #12604 For Research Use Only. Not For Use In Diagnostic Procedures. Entrez-Gene ID #10614 UniProt ID #O94992 Storage: Supplied in 10 mM sodium HEPES (pH 7.5), 150 Applications Species Cross-Reactivity* Molecular Wt. Isotype mM NaCl, 100 µg/ml BSA, 50% glycerol and less than 0.02% W, IP, IF-IC H, Mk 60 kDa Rabbit IgG** sodium azide. Store at –20°C. Do not aliquot the antibody. Endogenous *Species cross-reactivity is determined by western blot. Background: Hexamethylene bis-acetamide-inducible ** Anti-rabbit secondary antibodies must be used to protein 1 (HEXIM1) was originally identified in vascular kDa HeLa A-431 Hep G2 COS-7 detect this antibody. smooth muscle cells as a protein that is upregulated upon 200 Recommended Antibody Dilutions: treatment with the differentiating agent hexamethylene bi- 140 Western blotting 1:1000 sacetamide (1). HEXIM1 binds 7SK RNA, a highly abundant 100 Immunoprecipitation 1:100 non-coding RNA, and together they act as a potent inhibitor 80 Immunofluorescence (IF-IC) 1:1200 of positive transcription elongation factor b (P-TEFb) (2,3). 60 HEXIM1 P-TEFb phosphorylates the C-terminal domain of the largest 50 For product specific protocols please see the web page subunit of RNA polymerase II and is an important regulator for this product at www.cellsignal.com. 40 of transcription elongation (4-8). -
Protein Interaction Network of Alternatively Spliced Isoforms from Brain Links Genetic Risk Factors for Autism
ARTICLE Received 24 Aug 2013 | Accepted 14 Mar 2014 | Published 11 Apr 2014 DOI: 10.1038/ncomms4650 OPEN Protein interaction network of alternatively spliced isoforms from brain links genetic risk factors for autism Roser Corominas1,*, Xinping Yang2,3,*, Guan Ning Lin1,*, Shuli Kang1,*, Yun Shen2,3, Lila Ghamsari2,3,w, Martin Broly2,3, Maria Rodriguez2,3, Stanley Tam2,3, Shelly A. Trigg2,3,w, Changyu Fan2,3, Song Yi2,3, Murat Tasan4, Irma Lemmens5, Xingyan Kuang6, Nan Zhao6, Dheeraj Malhotra7, Jacob J. Michaelson7,w, Vladimir Vacic8, Michael A. Calderwood2,3, Frederick P. Roth2,3,4, Jan Tavernier5, Steve Horvath9, Kourosh Salehi-Ashtiani2,3,w, Dmitry Korkin6, Jonathan Sebat7, David E. Hill2,3, Tong Hao2,3, Marc Vidal2,3 & Lilia M. Iakoucheva1 Increased risk for autism spectrum disorders (ASD) is attributed to hundreds of genetic loci. The convergence of ASD variants have been investigated using various approaches, including protein interactions extracted from the published literature. However, these datasets are frequently incomplete, carry biases and are limited to interactions of a single splicing isoform, which may not be expressed in the disease-relevant tissue. Here we introduce a new interactome mapping approach by experimentally identifying interactions between brain-expressed alternatively spliced variants of ASD risk factors. The Autism Spliceform Interaction Network reveals that almost half of the detected interactions and about 30% of the newly identified interacting partners represent contribution from splicing variants, emphasizing the importance of isoform networks. Isoform interactions greatly contribute to establishing direct physical connections between proteins from the de novo autism CNVs. Our findings demonstrate the critical role of spliceform networks for translating genetic knowledge into a better understanding of human diseases. -
The Landscape of Somatic Mutations in Epigenetic Regulators Across 1,000 Paediatric Cancer Genomes
ARTICLE Received 24 Sep 2013 | Accepted 12 Mar 2014 | Published 8 Apr 2014 DOI: 10.1038/ncomms4630 The landscape of somatic mutations in epigenetic regulators across 1,000 paediatric cancer genomes Robert Huether1,*, Li Dong2,*, Xiang Chen1, Gang Wu1, Matthew Parker1, Lei Wei1, Jing Ma2, Michael N. Edmonson1, Erin K. Hedlund1, Michael C. Rusch1, Sheila A. Shurtleff2, Heather L. Mulder3, Kristy Boggs3, Bhavin Vadordaria3, Jinjun Cheng2, Donald Yergeau3, Guangchun Song2, Jared Becksfort1, Gordon Lemmon1, Catherine Weber2, Zhongling Cai2, Jinjun Dang2, Michael Walsh4, Amanda L. Gedman2, Zachary Faber2, John Easton3, Tanja Gruber2,4, Richard W. Kriwacki5, Janet F. Partridge6, Li Ding7,8,9, Richard K. Wilson7,8,9, Elaine R. Mardis7,8,9, Charles G. Mullighan2, Richard J. Gilbertson10, Suzanne J. Baker10, Gerard Zambetti6, David W. Ellison2, Jinghui Zhang1 & James R. Downing2 Studies of paediatric cancers have shown a high frequency of mutation across epigenetic regulators. Here we sequence 633 genes, encoding the majority of known epigenetic regulatory proteins, in over 1,000 paediatric tumours to define the landscape of somatic mutations in epigenetic regulators in paediatric cancer. Our results demonstrate a marked variation in the frequency of gene mutations across 21 different paediatric cancer subtypes, with the highest frequency of mutations detected in high-grade gliomas, T-lineage acute lymphoblastic leukaemia and medulloblastoma, and a paucity of mutations in low-grade glioma and retinoblastoma. The most frequently mutated genes are H3F3A, PHF6, ATRX, KDM6A, SMARCA4, ASXL2, CREBBP, EZH2, MLL2, USP7, ASXL1, NSD2, SETD2, SMC1A and ZMYM3. We identify novel loss-of-function mutations in the ubiquitin-specific processing protease 7 (USP7) in paediatric leukaemia, which result in decreased deubiquitination activity. -
Epigenome-Wide Exploratory Study of Monozygotic Twins Suggests Differentially Methylated Regions to Associate with Hand Grip Strength
Biogerontology (2019) 20:627–647 https://doi.org/10.1007/s10522-019-09818-1 (0123456789().,-volV)( 0123456789().,-volV) RESEARCH ARTICLE Epigenome-wide exploratory study of monozygotic twins suggests differentially methylated regions to associate with hand grip strength Mette Soerensen . Weilong Li . Birgit Debrabant . Marianne Nygaard . Jonas Mengel-From . Morten Frost . Kaare Christensen . Lene Christiansen . Qihua Tan Received: 15 April 2019 / Accepted: 24 June 2019 / Published online: 28 June 2019 Ó The Author(s) 2019 Abstract Hand grip strength is a measure of mus- significant CpG sites or pathways were found, how- cular strength and is used to study age-related loss of ever two of the suggestive top CpG sites were mapped physical capacity. In order to explore the biological to the COL6A1 and CACNA1B genes, known to be mechanisms that influence hand grip strength varia- related to muscular dysfunction. By investigating tion, an epigenome-wide association study (EWAS) of genomic regions using the comb-p algorithm, several hand grip strength in 672 middle-aged and elderly differentially methylated regions in regulatory monozygotic twins (age 55–90 years) was performed, domains were identified as significantly associated to using both individual and twin pair level analyses, the hand grip strength, and pathway analyses of these latter controlling the influence of genetic variation. regions revealed significant pathways related to the Moreover, as measurements of hand grip strength immune system, autoimmune disorders, including performed over 8 years were available in the elderly diabetes type 1 and viral myocarditis, as well as twins (age 73–90 at intake), a longitudinal EWAS was negative regulation of cell differentiation. -
Mutation and Expression Analysis in Medulloblastoma Yields Prognostic
Bien-Willner and Mitra Acta Neuropathologica Communications 2014, 2:74 http://www.actaneurocomms.org/content/2/1/74 RESEARCH Open Access Mutation and expression analysis in medulloblastoma yields prognostic variants and a putative mechanism of disease for i17q tumors Gabriel A Bien-Willner1,2* and Robi D Mitra2 Abstract Current consensus identifies four molecular subtypes of medulloblastoma (MB): WNT, sonic hedgehog (SHH), and groups “3/C” and “4/D”. Group 4 is not well characterized, but harbors the most frequently observed chromosomal abnormality in MB, i17q, whose presence may confer a worse outcome. Recent publications have identified mutations in chromatin remodeling genes that may be overrepresented in this group, suggesting a biological role for these genes in i17q. This work seeks to explore the pathology that underlies i17q in MB. Specifically, we examine the prognostic significance of the previously-identified gene mutations in an independent set of MBs as well as to examine biological relevance of these genes and related pathways by gene expression profiling. The previously-implicated p53 signaling pathway is also examined as a putative driver of i17q tumor oncogenesis. The data show gene mutations associated with i17q tumors in previous studies (KMD6A, ZMYM3, MLL3 and GPS2) were correlated with significantly worse outcomes despite not being specific to i17q in this set. Expression of these genes did not appear to underlie the biology of the molecular variants. TP53 expression was significantly reduced in i17q/ group 4 tumors; this could not be accounted for by dosage effects alone. Expression of regulators and mediators of p53 signaling were significantly altered in i17q tumors. -
Molecular Effects of Isoflavone Supplementation Human Intervention Studies and Quantitative Models for Risk Assessment
Molecular effects of isoflavone supplementation Human intervention studies and quantitative models for risk assessment Vera van der Velpen Thesis committee Promotors Prof. Dr Pieter van ‘t Veer Professor of Nutritional Epidemiology Wageningen University Prof. Dr Evert G. Schouten Emeritus Professor of Epidemiology and Prevention Wageningen University Co-promotors Dr Anouk Geelen Assistant professor, Division of Human Nutrition Wageningen University Dr Lydia A. Afman Assistant professor, Division of Human Nutrition Wageningen University Other members Prof. Dr Jaap Keijer, Wageningen University Dr Hubert P.J.M. Noteborn, Netherlands Food en Consumer Product Safety Authority Prof. Dr Yvonne T. van der Schouw, UMC Utrecht Dr Wendy L. Hall, King’s College London This research was conducted under the auspices of the Graduate School VLAG (Advanced studies in Food Technology, Agrobiotechnology, Nutrition and Health Sciences). Molecular effects of isoflavone supplementation Human intervention studies and quantitative models for risk assessment Vera van der Velpen Thesis submitted in fulfilment of the requirements for the degree of doctor at Wageningen University by the authority of the Rector Magnificus Prof. Dr M.J. Kropff, in the presence of the Thesis Committee appointed by the Academic Board to be defended in public on Friday 20 June 2014 at 13.30 p.m. in the Aula. Vera van der Velpen Molecular effects of isoflavone supplementation: Human intervention studies and quantitative models for risk assessment 154 pages PhD thesis, Wageningen University, Wageningen, NL (2014) With references, with summaries in Dutch and English ISBN: 978-94-6173-952-0 ABSTRact Background: Risk assessment can potentially be improved by closely linked experiments in the disciplines of epidemiology and toxicology. -
Novel Driver Strength Index Highlights Important Cancer Genes in TCGA Pancanatlas Patients
medRxiv preprint doi: https://doi.org/10.1101/2021.08.01.21261447; this version posted August 5, 2021. The copyright holder for this preprint (which was not certified by peer review) is the author/funder, who has granted medRxiv a license to display the preprint in perpetuity. It is made available under a CC-BY-NC-ND 4.0 International license . Novel Driver Strength Index highlights important cancer genes in TCGA PanCanAtlas patients Aleksey V. Belikov*, Danila V. Otnyukov, Alexey D. Vyatkin and Sergey V. Leonov Laboratory of Innovative Medicine, School of Biological and Medical Physics, Moscow Institute of Physics and Technology, 141701 Dolgoprudny, Moscow Region, Russia *Corresponding author: [email protected] NOTE: This preprint reports new research that has not been certified by peer review and should not be used to guide clinical practice. 1 medRxiv preprint doi: https://doi.org/10.1101/2021.08.01.21261447; this version posted August 5, 2021. The copyright holder for this preprint (which was not certified by peer review) is the author/funder, who has granted medRxiv a license to display the preprint in perpetuity. It is made available under a CC-BY-NC-ND 4.0 International license . Abstract Elucidating crucial driver genes is paramount for understanding the cancer origins and mechanisms of progression, as well as selecting targets for molecular therapy. Cancer genes are usually ranked by the frequency of mutation, which, however, does not necessarily reflect their driver strength. Here we hypothesize that driver strength is higher for genes that are preferentially mutated in patients with few driver mutations overall, because these few mutations should be strong enough to initiate cancer. -
The Function and Evolution of C2H2 Zinc Finger Proteins and Transposons
The function and evolution of C2H2 zinc finger proteins and transposons by Laura Francesca Campitelli A thesis submitted in conformity with the requirements for the degree of Doctor of Philosophy Department of Molecular Genetics University of Toronto © Copyright by Laura Francesca Campitelli 2020 The function and evolution of C2H2 zinc finger proteins and transposons Laura Francesca Campitelli Doctor of Philosophy Department of Molecular Genetics University of Toronto 2020 Abstract Transcription factors (TFs) confer specificity to transcriptional regulation by binding specific DNA sequences and ultimately affecting the ability of RNA polymerase to transcribe a locus. The C2H2 zinc finger proteins (C2H2 ZFPs) are a TF class with the unique ability to diversify their DNA-binding specificities in a short evolutionary time. C2H2 ZFPs comprise the largest class of TFs in Mammalian genomes, including nearly half of all Human TFs (747/1,639). Positive selection on the DNA-binding specificities of C2H2 ZFPs is explained by an evolutionary arms race with endogenous retroelements (EREs; copy-and-paste transposable elements), where the C2H2 ZFPs containing a KRAB repressor domain (KZFPs; 344/747 Human C2H2 ZFPs) are thought to diversify to bind new EREs and repress deleterious transposition events. However, evidence of the gain and loss of KZFP binding sites on the ERE sequence is sparse due to poor resolution of ERE sequence evolution, despite the recent publication of binding preferences for 242/344 Human KZFPs. The goal of my doctoral work has been to characterize the Human C2H2 ZFPs, with specific interest in their evolutionary history, functional diversity, and coevolution with LINE EREs. -
Supplementary Table 3: Genes Only Influenced By
Supplementary Table 3: Genes only influenced by X10 Illumina ID Gene ID Entrez Gene Name Fold change compared to vehicle 1810058M03RIK -1.104 2210008F06RIK 1.090 2310005E10RIK -1.175 2610016F04RIK 1.081 2610029K11RIK 1.130 381484 Gm5150 predicted gene 5150 -1.230 4833425P12RIK -1.127 4933412E12RIK -1.333 6030458P06RIK -1.131 6430550H21RIK 1.073 6530401D06RIK 1.229 9030607L17RIK -1.122 A330043C08RIK 1.113 A330043L12 1.054 A530092L01RIK -1.069 A630054D14 1.072 A630097D09RIK -1.102 AA409316 FAM83H family with sequence similarity 83, member H 1.142 AAAS AAAS achalasia, adrenocortical insufficiency, alacrimia 1.144 ACADL ACADL acyl-CoA dehydrogenase, long chain -1.135 ACOT1 ACOT1 acyl-CoA thioesterase 1 -1.191 ADAMTSL5 ADAMTSL5 ADAMTS-like 5 1.210 AFG3L2 AFG3L2 AFG3 ATPase family gene 3-like 2 (S. cerevisiae) 1.212 AI256775 RFESD Rieske (Fe-S) domain containing 1.134 Lipo1 (includes AI747699 others) lipase, member O2 -1.083 AKAP8L AKAP8L A kinase (PRKA) anchor protein 8-like -1.263 AKR7A5 -1.225 AMBP AMBP alpha-1-microglobulin/bikunin precursor 1.074 ANAPC2 ANAPC2 anaphase promoting complex subunit 2 -1.134 ANKRD1 ANKRD1 ankyrin repeat domain 1 (cardiac muscle) 1.314 APOA1 APOA1 apolipoprotein A-I -1.086 ARHGAP26 ARHGAP26 Rho GTPase activating protein 26 -1.083 ARL5A ARL5A ADP-ribosylation factor-like 5A -1.212 ARMC3 ARMC3 armadillo repeat containing 3 -1.077 ARPC5 ARPC5 actin related protein 2/3 complex, subunit 5, 16kDa -1.190 activating transcription factor 4 (tax-responsive enhancer element ATF4 ATF4 B67) 1.481 AU014645 NCBP1 nuclear cap -
Efficacy of Cyclin-Dependent-Kinase 9 Inhibitors in a Murine Model Of
Leukemia (2014) 28, 1427–1435 & 2014 Macmillan Publishers Limited All rights reserved 0887-6924/14 www.nature.com/leu ORIGINAL ARTICLE Efficacy of cyclin-dependent-kinase 9 inhibitors in a murine model of mixed-lineage leukemia M-P Garcia-Cuellar1,EFu¨ ller1,EMa¨thner1, C Breitinger1, K Hetzner1, L Zeitlmann2, A Borkhardt3 and RK Slany1 Mixed-lineage leukemia fusion proteins activate their target genes predominantly by stimulating transcriptional elongation. A core component necessary for this activity is cyclin-dependent kinase 9. Here we explored the effectiveness of small molecules targeting this enzyme as potential therapeutics. A screen of seven compounds with anti-CDK9 activity applied to a panel of leukemia cell lines identified flavopiridol and the experimental inhibitor PC585 as superior in efficacy with inhibitory concentrations in the submicromolar range. Both substances induced rapid dephosphorylation of the RNA polymerase II C-terminal domain, accompanied by downregulation of CDK9-dependent transcripts for MYC and HOXA9. Global gene expression analysis indicated the induction of a general stress response program, culminating in widespread apoptosis. Importantly, colony-forming activity in leukemia lines and primary patient samples could be completely inhibited under conditions that did not affect native precursors from bone marrow. In vivo application in a mouse transplant model significantly delayed disease with PC585 showing also oral activity. These results suggest CDK9 inhibition as novel treatment option for mixed-lineage leukemia. Leukemia (2014) 28, 1427–1435; doi:10.1038/leu.2014.40 Keywords: mixed-lineage leukemia; CDK9; inhibitor; preclinical study INTRODUCTION complex) including P-TEFb is responsible for elongation control,5 Despite considerable progress in leukemia treatment, mixed- DotCom (DOT1L complex) methylates histone H3 at lysine 79 lineage leukemia (MLL) remains a disease with a very dismal through the catalytic activity of the histone methyltransferase 12 prognosis. -
Whole Exome Sequencing Reveals NOTCH1 Mutations in Anaplastic Large Cell Lymphoma and Points to Notch Both As a Key Pathway and a Potential Therapeutic Target
Non-Hodgkin Lymphoma SUPPLEMENTARY APPENDIX Whole exome sequencing reveals NOTCH1 mutations in anaplastic large cell lymphoma and points to Notch both as a key pathway and a potential therapeutic target Hugo Larose, 1,2 Nina Prokoph, 1,2 Jamie D. Matthews, 1 Michaela Schlederer, 3 Sandra Högler, 4 Ali F. Alsulami, 5 Stephen P. Ducray, 1,2 Edem Nuglozeh, 6 Mohammad Feroze Fazaludeen, 7 Ahmed Elmouna, 6 Monica Ceccon, 2,8 Luca Mologni, 2,8 Carlo Gambacorti-Passerini, 2,8 Gerald Hoefler, 9 Cosimo Lobello, 2,10 Sarka Pospisilova, 2,10,11 Andrea Janikova, 2,11 Wilhelm Woessmann, 2,12 Christine Damm-Welk, 2,12 Martin Zimmermann, 13 Alina Fedorova, 14 Andrea Malone, 15 Owen Smith, 15 Mariusz Wasik, 2,16 Giorgio Inghirami, 17 Laurence Lamant, 18 Tom L. Blundell, 5 Wolfram Klapper, 19 Olaf Merkel, 2,3 G. A. Amos Burke, 20 Shahid Mian, 6 Ibraheem Ashankyty, 21 Lukas Kenner 2,3,22 and Suzanne D. Turner 1,2,10 1Department of Pathology, University of Cambridge, Cambridge, UK; 2European Research Initiative for ALK Related Malignancies (ERIA; www.ERIALCL.net ); 3Department of Pathology, Medical University of Vienna, Vienna, Austria; 4Unit of Laboratory Animal Pathology, Uni - versity of Veterinary Medicine Vienna, Vienna, Austria; 5Department of Biochemistry, University of Cambridge, Tennis Court Road, Cam - bridge, UK; 6Molecular Diagnostics and Personalised Therapeutics Unit, Colleges of Medicine and Applied Medical Sciences, University of Ha’il, Ha’il, Saudi Arabia; 7Neuroinflammation Research Group, Department of Neurobiology, A.I Virtanen Institute -
Beyond Gene Expression
Beyond gene expression Citation for published version (APA): Gupta, R. (2021). Beyond gene expression: novel methods and applications of transcript expression analyses in RNA-Seq. Maastricht University. https://doi.org/10.26481/dis.20210304rg Document status and date: Published: 01/01/2021 DOI: 10.26481/dis.20210304rg Document Version: Publisher's PDF, also known as Version of record Please check the document version of this publication: • A submitted manuscript is the version of the article upon submission and before peer-review. There can be important differences between the submitted version and the official published version of record. People interested in the research are advised to contact the author for the final version of the publication, or visit the DOI to the publisher's website. • The final author version and the galley proof are versions of the publication after peer review. • The final published version features the final layout of the paper including the volume, issue and page numbers. Link to publication General rights Copyright and moral rights for the publications made accessible in the public portal are retained by the authors and/or other copyright owners and it is a condition of accessing publications that users recognise and abide by the legal requirements associated with these rights. • Users may download and print one copy of any publication from the public portal for the purpose of private study or research. • You may not further distribute the material or use it for any profit-making activity or commercial gain • You may freely distribute the URL identifying the publication in the public portal.