Structural Evolution of the Rio Grande Rift
Total Page:16
File Type:pdf, Size:1020Kb
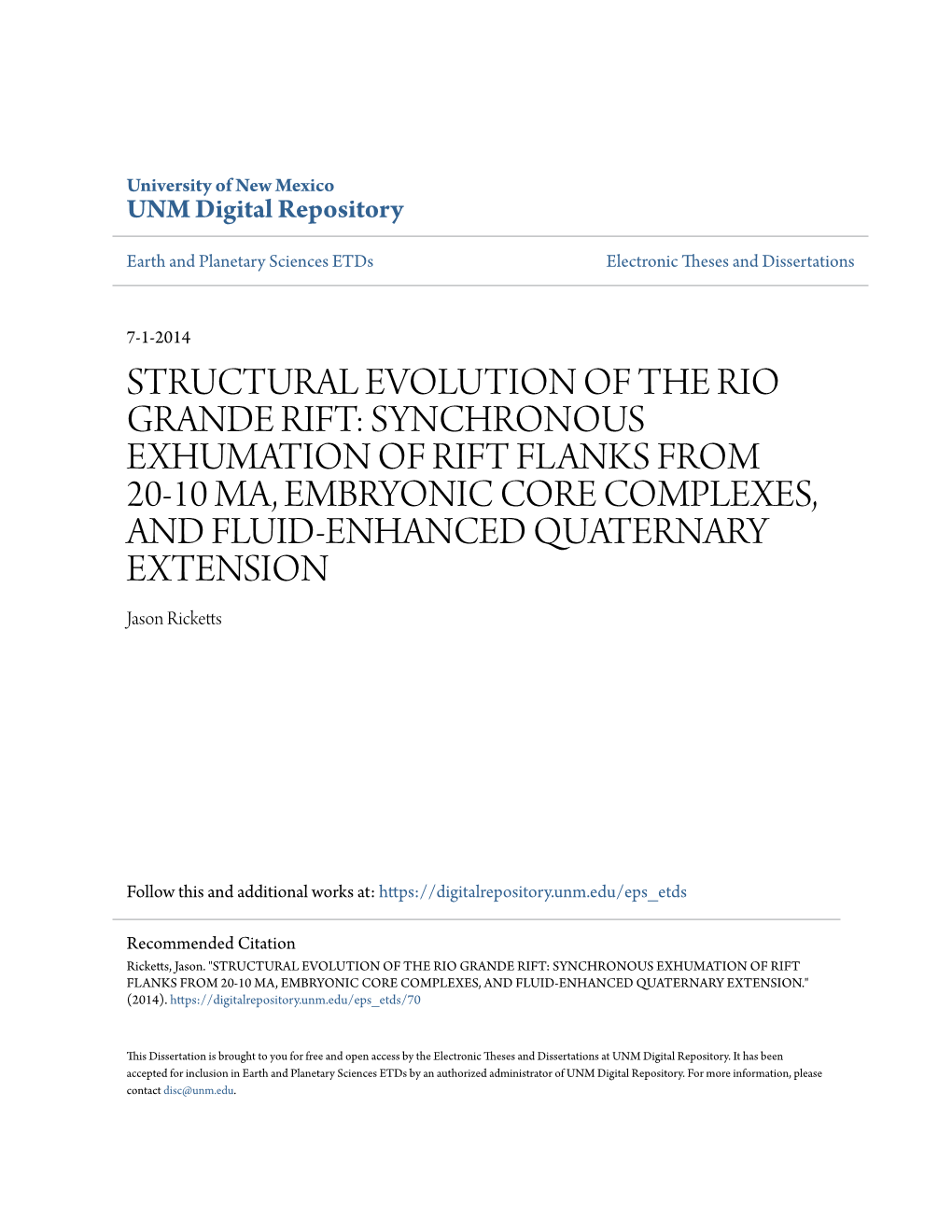
Load more
Recommended publications
-
An Interpretation of the Structural Geology of the Franklin Mountains, Texas Earl M
New Mexico Geological Society Downloaded from: http://nmgs.nmt.edu/publications/guidebooks/26 An interpretation of the structural geology of the Franklin Mountains, Texas Earl M. P. Lovejoy, 1975, pp. 261-268 in: Las Cruces Country, Seager, W. R.; Clemons, R. E.; Callender, J. F.; [eds.], New Mexico Geological Society 26th Annual Fall Field Conference Guidebook, 376 p. This is one of many related papers that were included in the 1975 NMGS Fall Field Conference Guidebook. Annual NMGS Fall Field Conference Guidebooks Every fall since 1950, the New Mexico Geological Society (NMGS) has held an annual Fall Field Conference that explores some region of New Mexico (or surrounding states). Always well attended, these conferences provide a guidebook to participants. Besides detailed road logs, the guidebooks contain many well written, edited, and peer-reviewed geoscience papers. These books have set the national standard for geologic guidebooks and are an essential geologic reference for anyone working in or around New Mexico. Free Downloads NMGS has decided to make peer-reviewed papers from our Fall Field Conference guidebooks available for free download. Non-members will have access to guidebook papers two years after publication. Members have access to all papers. This is in keeping with our mission of promoting interest, research, and cooperation regarding geology in New Mexico. However, guidebook sales represent a significant proportion of our operating budget. Therefore, only research papers are available for download. Road logs, mini-papers, maps, stratigraphic charts, and other selected content are available only in the printed guidebooks. Copyright Information Publications of the New Mexico Geological Society, printed and electronic, are protected by the copyright laws of the United States. -
Bilek Earth and Environmental Sciences Dept
Susan L. Bilek Earth and Environmental Sciences Dept. New Mexico Institute of Mining and Technology, Socorro, NM 87801 Email: [email protected] Webpage: https://nmt.edu/academics/ees/faculty/sbilek.php Professional Preparation: B.S., with honors, Geosciences, minor in Economics, Penn State University, May 1996 M.S., Earth Sciences/Geophysics, University of California, Santa Cruz, June 1998 M.S. Thesis title: Lower mantle heterogeneity beneath Eurasia imaged by parametric migration of shear waves Ph.D. Earth Sciences/Geophysics, University of California, Santa Cruz, June 2001 Ph.D. Dissertation title: Multi-scale examination of seismogenic zone processes in circum- Pacific subduction zones Turner Postdoctoral Fellow, Dept. Geological Sciences, University of Michigan, 2001-2003 Appointments: Professor, Earth and Environmental Science Dept., New Mexico Tech, 2014 – present Associate Professor, Earth and Environmental Sciences Dept., New Mexico Tech, 2008 – 2014 Assistant Professor, Earth and Environmental Sciences Dept., New Mexico Tech, 2003 – 2008 Selected Recent Synergistic Activities: National/International: Board of Directors, Seismological Society of America, 2020-2022 Associate Editor, Geophysical Research Letters, 2020-present Editorial Board, Tectonophysics, 2016-present Associate Guest Editor, Subduction Top to Bottom 2, special issue of Geospheres, 2016-present PI of IRIS PASSCAL Instrument Center, Oct 2013-present IRIS Board of Directors, 2010-2012, Board secretary 2012 Reviewer: NSF, NNSA, Science, Science Advances, Nature, -
Slip Rate of the Western Garlock Fault, at Clark Wash, Near Lone Tree Canyon, Mojave Desert, California
Slip rate of the western Garlock fault, at Clark Wash, near Lone Tree Canyon, Mojave Desert, California Sally F. McGill1†, Stephen G. Wells2, Sarah K. Fortner3*, Heidi Anderson Kuzma1**, John D. McGill4 1Department of Geological Sciences, California State University, San Bernardino, 5500 University Parkway, San Bernardino, California 92407-2397, USA 2Desert Research Institute, PO Box 60220, Reno, Nevada 89506-0220, USA 3Department of Geology and Geophysics, University of Wisconsin-Madison, 1215 W Dayton St., Madison, Wisconsin 53706, USA 4Department of Physics, California State University, San Bernardino, 5500 University Parkway, San Bernardino, California 92407-2397, USA *Now at School of Earth Sciences, The Ohio State University, 275 Mendenhall Laboratory, 125 S. Oval Mall, Columbus, Ohio 43210, USA **Now at Department of Civil and Environmental Engineering, 760 Davis Hall, University of California, Berkeley, California, 94720-1710, USA ABSTRACT than rates inferred from geodetic data. The ously published slip-rate estimates from a simi- high rate of motion on the western Garlock lar time period along the central section of the The precise tectonic role of the left-lateral fault is most consistent with a model in which fault (Clark and Lajoie, 1974; McGill and Sieh, Garlock fault in southern California has the western Garlock fault acts as a conju- 1993). This allows us to assess how the slip rate been controversial. Three proposed tectonic gate shear to the San Andreas fault. Other changes as a function of distance along strike. models yield signifi cantly different predic- mechanisms, involving extension north of the Our results also fi ll an important temporal niche tions for the slip rate, history, orientation, Garlock fault and block rotation at the east- between slip rates estimated at geodetic time and total bedrock offset as a function of dis- ern end of the fault may be relevant to the scales (past decade or two) and fault motions tance along strike. -
An Environmental History of the Middle Rio Grande Basin
United States Department of From the Rio to the Sierra: Agriculture Forest Service An Environmental History of Rocky Mountain Research Station the Middle Rio Grande Basin Fort Collins, Colorado 80526 General Technical Report RMRS-GTR-5 Dan Scurlock i Scurlock, Dan. 1998. From the rio to the sierra: An environmental history of the Middle Rio Grande Basin. General Technical Report RMRS-GTR-5. Fort Collins, CO: U.S. Department of Agriculture, Forest Service, Rocky Mountain Research Station. 440 p. Abstract Various human groups have greatly affected the processes and evolution of Middle Rio Grande Basin ecosystems, especially riparian zones, from A.D. 1540 to the present. Overgrazing, clear-cutting, irrigation farming, fire suppression, intensive hunting, and introduction of exotic plants have combined with droughts and floods to bring about environmental and associated cultural changes in the Basin. As a result of these changes, public laws were passed and agencies created to rectify or mitigate various environmental problems in the region. Although restoration and remedial programs have improved the overall “health” of Basin ecosystems, most old and new environmental problems persist. Keywords: environmental impact, environmental history, historic climate, historic fauna, historic flora, Rio Grande Publisher’s Note The opinions and recommendations expressed in this report are those of the author and do not necessarily reflect the views of the USDA Forest Service. Mention of trade names does not constitute endorsement or recommendation for use by the Federal Government. The author withheld diacritical marks from the Spanish words in text for consistency with English punctuation. Publisher Rocky Mountain Research Station Fort Collins, Colorado May 1998 You may order additional copies of this publication by sending your mailing information in label form through one of the following media. -
Map 3.1 Travel Management Plan
TOADLENA SHEEP SPRINGS GREAT BENDTHE PILLAR 3 NW THE PILLAR 3 NE TANNER LAKE PRETTY ROCKPUEBLO BONITO NW B836108 B636108 B536108 B436108 KIMBETO LYBROOK NW LYBROOK COUNSELORTANCOSA WINDMILL B336108 B236108 B136108 FIVE LAKES CANYON NEREGINA GALLINA ARROYA DEL AGUAYOUNGSVILLE CANONES ABIQUIU Y 134 B836107 B736107 B636107 B536107 B436107 MEDANALES LYDEN VELARDE TRAMPAS PENASCO TRES RITOS CERRO VISTA CHACON W B336107 B136107 B836106 B736106 B636106 B536106 E H B436106 B336106 B236106 B136106 B836105 B736105 B636105 B536105 B436105 B336105 T TOADLENA FIVE LAKES CANYON NW U STA 8 S 6 SONSELA BUTTES B236107 CRYSTAL WASHINGTON PASS S H Y A136109 NASCHITTI CHACO CANYON T W W A836108 THE PILLAR 3 SW A H A736108 THE PILLAR 3 SE Y E A636108 LA VIDA MISSION T T E A436108 PUEBLO BONITO HW 8 A A336108 FIRE ROCK WELL Y 44 ABIQUIU 4 TAOS GREY HILL SPRING T A236108 A836107 LYBROOK SE MULE DAM DEER MESA A636107 TAYLOR RANCH CUBA S A536108 KIN KLIZHIN RUINS A536107 A436107 A336107 NACIMIENTO PEAK JAROSA POLVADERA PEAK SARGENT RANCH A236107 A836106 VALLECITOS CHILI SAN JUAN PUEBLO CHIMAYO TRUCHAS EL VALLE JICARITA PEAK HOLMAN A136108 A736106 A636106 A436106 COMANCHE PEAK A736107 ARROYO CHIJUILLITA A336106 A236106 A136106 A836105 A736105 A636105 A536105 A436105 S A336105 CERRO DEL GRANT 6 A136107 7 T A536106 Y A W T STATE H HWY STAT E E BUELL PARK TODILTO PARK 1 H CHUSKA PEAK 26 W H135109 H835108 EAR ROCK Y H735108 RED LAKE WELL 5 H535108 MILK LAKE NOSE ROCK 18 COYOTE CANYON NW H335108 SEVEN LAKES NEPUEBLO PINTADO STANDING ROCK NW H235108 H135108 STAR LAKE -
Ground-Water Geochemistry of the Albuquerque-Belen Basin, Central New Mexico
GROUND-WA TER GEOCHEMISTRY OF THE ALBVQVERQVE-BELEN BASIN, CENTRAL NEW MEXICO By Scott K. Anderholm U.S. GEOLOGICAL SURVEY Water-Resources Investigations Report 86-4094 Albuquerque, New Mexico 1988 DEPARTMENT OF THE INTERIOR DONALD PAUL MODEL, Secretary U.S. GEOLOGICAL SURVEY Dallas L. Peck, Director For additional information Copies of this report can write to: be purchased from: District Chief U.S. Geological Survey U.S. Geological Survey Water Resources Division Books and Open-File Reports Pinetree Office Park Federal Center, Building 810 4501 Indian School Rd. NE, Suite 200 Box 25425 Albuquerque, New Mexico 87110 Denver, Colorado 80225 CONTENTS Page Abstract ............................................................. 1 Introduction ......................................................... 2 Acknowledgments ................................................. 4 Purpose and scope ............................................... 4 Location ........................................................ 4 Climate ......................................................... 6 Previous investigations ......................................... 6 Geology .................................................... 6 Hydrology .................................................. 6 Well-numbering system ........................................... 9 Geology .............................................................. 10 Precambrian rocks ............................................... 10 Paleozoic rocks ................................................. 10 Mesozoic -
The Geology of the Enosburg Area, Vermont
THE GEOLOGY OF THE ENOSBURG AREA, VERMONT By JOlIN G. DENNIS VERMONT GEOLOGICAL SURVEY CHARLES G. DOLL, State Geologist Published by VERMONT DEVELOPMENT DEPARTMENT MONTPELIER, VERMONT BULLETIN No. 23 1964 TABLE OF CONTENTS PAGE ABSTRACT 7 INTRODUCTION ...................... 7 Location ........................ 7 Geologic Setting .................... 9 Previous Work ..................... 10 Method of Study .................... 10 Acknowledgments .................... 10 Physiography ...................... 11 STRATIGRAPHY ...................... 12 Introduction ...................... 12 Pinnacle Formation ................... 14 Name and Distribution ................ 14 Graywacke ...................... 14 Underhill Facics ................... 16 Tibbit Hill Volcanics ................. 16 Age......................... 19 Underhill Formation ................... 19 Name and Distribution ................ 19 Fairfield Pond Member ................ 20 White Brook Member ................. 21 West Sutton Slate ................... 22 Bonsecours Facies ................... 23 Greenstones ..................... 24 Stratigraphic Relations of the Greenstones ........ 25 Cheshire Formation ................... 26 Name and Distribution ................ 26 Lithology ...................... 26 Age......................... 27 Bridgeman Hill Formation ................ 28 Name and Distribution ................ 28 Dunham Dolomite .................. 28 Rice Hill Member ................... 29 Oak Hill Slate (Parker Slate) .............. 29 Rugg Brook Dolomite (Scottsmore -
Geology of the Cebolla Quadrangle, Rio Arriba County, New Mexico
BULLETIN 92 Geology of the Cebolla Quadrangle Rio Arriba County, New Mexico by HUGH H. DONEY 1 9 6 8 STATE BUREAU OF MINES AND MINERAL RESOURCES NEW MEXICO INSTITUTE OF MINING & TECHNOLOGY CAMPUS STATION SOCORRO, NEW MEXICO NEW MEXICO INSTITUTE OF MINING AND TECHNOLOGY STIRLING A. COLGATE, President STATE BUREAU OF MINES AND MINERAL RESOURCES FRANK E. KOTTLOWSKI, Acting Director THE REGENTS MEMBERS Ex OFFICIO The Honorable David F. Cargo ...................................... Governor of New Mexico Leonard DeLayo ................................................. Superintendent of Public Instruction APPOINTED MEMBERS William G. Abbott .........................................................................................Hobbs Henry S. Birdseye ............................................................................... Albuquerque Thomas M. Cramer ................................................................................... Carlsbad Steve S. Torres, Jr. ....................................................................................... Socorro Richard M. Zimmerly .................................................................................... Socorro For sale by the New Mexico Bureau of Mines and Mineral Resources Campus Station, Socorro, N. Mex. 87801—Price $3.00 Abstract The Cebolla quadrangle overlaps two physiographic provinces, the San Juan Basin and the Tusas Mountains. Westward-dipping Mesozoic rocks, Quaternary cinder cones and flow rock, and Quaternary gravel terraces occur in the Chama Basin of the San Juan -
Kinematics of the Northern Walker Lane: an Incipient Transform Fault Along the Pacific–North American Plate Boundary
Kinematics of the northern Walker Lane: An incipient transform fault along the Paci®c±North American plate boundary James E. Faulds Christopher D. Henry Nevada Bureau of Mines and Geology, MS 178, University of Nevada, Reno, Nevada 89557, USA Nicholas H. Hinz ABSTRACT GEOLOGIC SETTING In the western Great Basin of North America, a system of dextral faults accommodates As western North America has evolved 15%±25% of the Paci®c±North American plate motion. The northern Walker Lane in from a convergent to a transform margin in northwest Nevada and northeast California occupies the northern terminus of this system. the past 30 m.y., the northern Walker Lane has This young evolving part of the plate boundary offers insight into how strike-slip fault undergone widespread volcanism and tecto- systems develop and may re¯ect the birth of a transform fault. A belt of overlapping, left- nism. Tertiary volcanic strata include 31±23 stepping dextral faults dominates the northern Walker Lane. Offset segments of a W- Ma ash-¯ow tuffs associated with the south- trending Oligocene paleovalley suggest ;20±30 km of cumulative dextral slip beginning ward-migrating ``ignimbrite ¯are up,'' 22±5 ca. 9±3 Ma. The inferred long-term slip rate of ;2±10 mm/yr is compatible with global Ma calc-alkaline intermediate-composition positioning system observations of the current strain ®eld. We interpret the left-stepping rocks related to the ancestral Cascade arc, and faults as macroscopic Riedel shears developing above a nascent lithospheric-scale trans- 13 Ma to present bimodal rocks linked to Ba- form fault. -
The East African Rift System in the Light of KRISP 90
ELSEVIER Tectonophysics 236 (1994) 465-483 The East African rift system in the light of KRISP 90 G.R. Keller a, C. Prodehl b, J. Mechie b,l, K. Fuchs b, M.A. Khan ‘, P.K.H. Maguire ‘, W.D. Mooney d, U. Achauer e, P.M. Davis f, R.P. Meyer g, L.W. Braile h, 1.0. Nyambok i, G.A. Thompson J a Department of Geological Sciences, University of Texas at El Paso, El Paso, TX 79968-0555, USA b Geophysikalisches Institut, Universitdt Karlwuhe, Hertzstrasse 16, D-76187Karlsruhe, Germany ’ Department of Geology, University of Leicester, University Road, Leicester LEl 7RH, UK d U.S. Geological Survey, Office of Earthquake Research, 345 Middlefield Road, Menlo Park, CA 94025, USA ’ Institut de Physique du Globe, Universite’ de Strasbourg, 5 Rue Ret& Descartes, F-67084 Strasbourg, France ‘Department of Earth and Space Sciences, University of California at Los Angeles, Los Angeles, CA 90024, USA ’ Department of Geology and Geophysics, University of Wuconsin at Madison, Madison, WI 53706, USA h Department of Earth and Atmospheric Sciences, Purdue University, West Lafayette, IN 47907, USA i Department of Geology, University of Nairobi, P.O. Box 14576, Nairobi, Kenya ’ Department of Geophysics, Stanford University, Stanford, CA 94305, USA Received 21 September 1992; accepted 8 November 1993 Abstract On the basis of a test experiment in 1985 (KRISP 85) an integrated seismic-refraction/ teleseismic survey (KRISP 90) was undertaken to study the deep structure beneath the Kenya rift down to depths of NO-150 km. This paper summarizes the highlights of KRISP 90 as reported in this volume and discusses their broad implications as well as the structure of the Kenya rift in the general framework of other continental rifts. -
Geologic Summary of the Abiquiu Quadrangle, North-Central New Mexico Florian Maldonado and Daniel P
New Mexico Geological Society Downloaded from: http://nmgs.nmt.edu/publications/guidebooks/58 Geologic summary of the Abiquiu quadrangle, north-central New Mexico Florian Maldonado and Daniel P. Miggins, 2007, pp. 182-187 in: Geology of the Jemez Region II, Kues, Barry S., Kelley, Shari A., Lueth, Virgil W.; [eds.], New Mexico Geological Society 58th Annual Fall Field Conference Guidebook, 499 p. This is one of many related papers that were included in the 2007 NMGS Fall Field Conference Guidebook. Annual NMGS Fall Field Conference Guidebooks Every fall since 1950, the New Mexico Geological Society (NMGS) has held an annual Fall Field Conference that explores some region of New Mexico (or surrounding states). Always well attended, these conferences provide a guidebook to participants. Besides detailed road logs, the guidebooks contain many well written, edited, and peer-reviewed geoscience papers. These books have set the national standard for geologic guidebooks and are an essential geologic reference for anyone working in or around New Mexico. Free Downloads NMGS has decided to make peer-reviewed papers from our Fall Field Conference guidebooks available for free download. Non-members will have access to guidebook papers two years after publication. Members have access to all papers. This is in keeping with our mission of promoting interest, research, and cooperation regarding geology in New Mexico. However, guidebook sales represent a significant proportion of our operating budget. Therefore, only research papers are available for download. Road logs, mini-papers, maps, stratigraphic charts, and other selected content are available only in the printed guidebooks. Copyright Information Publications of the New Mexico Geological Society, printed and electronic, are protected by the copyright laws of the United States. -
The Geology of New Mexico As Understood in 1912: an Essay for the Centennial of New Mexico Statehood Part 2 Barry S
Celebrating New Mexico's Centennial The geology of New Mexico as understood in 1912: an essay for the centennial of New Mexico statehood Part 2 Barry S. Kues, Department of Earth and Planetary Sciences, University of New Mexico, Albuquerque, New Mexico, [email protected] Introduction he first part of this contribution, presented in the February Here I first discuss contemporary ideas on two fundamental areas 2012 issue of New Mexico Geology, laid the groundwork for an of geologic thought—the accurate dating of rocks and the move- exploration of what geologists knew or surmised about the ment of continents through time—that were at the beginning of Tgeology of New Mexico as the territory transitioned into statehood paradigm shifts around 1912. Then I explore research trends and in 1912. Part 1 included an overview of the demographic, economic, the developing state of knowledge in stratigraphy and paleontol- social, cultural, and technological attributes of New Mexico and its ogy, two disciplines of geology that were essential in understand- people a century ago, and a discussion of important individuals, ing New Mexico’s rock record (some 84% of New Mexico’s surface institutions, and areas and methods of research—the geologic envi- area is covered by sediments or sedimentary rocks) and which were ronment, so to speak—that existed in the new state at that time. advancing rapidly through the first decade of the 20th century. The geologic time scale and age of rocks The geologic time scale familiar to geologists working in New The USGS did not adopt the Paleocene as the earliest epoch of the Mexico in 1912 was not greatly different from that used by modern Cenozoic until 1939.