Disrupted Circadian Core-Clock Oscillations in Type 2 Diabetes Are Linked to Altered Rhythmic Mitochondrial Metabolism
Total Page:16
File Type:pdf, Size:1020Kb
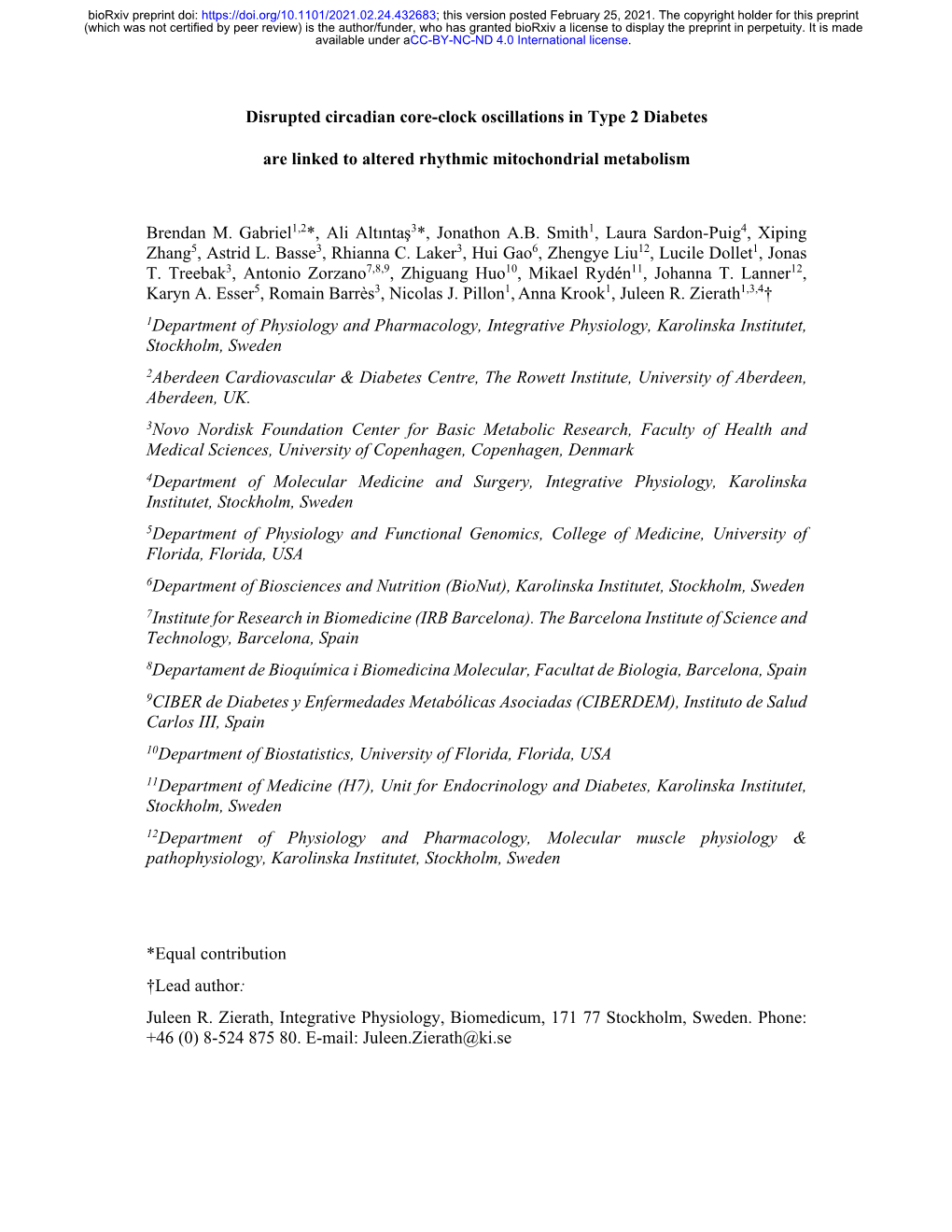
Load more
Recommended publications
-
A Computational Approach for Defining a Signature of Β-Cell Golgi Stress in Diabetes Mellitus
Page 1 of 781 Diabetes A Computational Approach for Defining a Signature of β-Cell Golgi Stress in Diabetes Mellitus Robert N. Bone1,6,7, Olufunmilola Oyebamiji2, Sayali Talware2, Sharmila Selvaraj2, Preethi Krishnan3,6, Farooq Syed1,6,7, Huanmei Wu2, Carmella Evans-Molina 1,3,4,5,6,7,8* Departments of 1Pediatrics, 3Medicine, 4Anatomy, Cell Biology & Physiology, 5Biochemistry & Molecular Biology, the 6Center for Diabetes & Metabolic Diseases, and the 7Herman B. Wells Center for Pediatric Research, Indiana University School of Medicine, Indianapolis, IN 46202; 2Department of BioHealth Informatics, Indiana University-Purdue University Indianapolis, Indianapolis, IN, 46202; 8Roudebush VA Medical Center, Indianapolis, IN 46202. *Corresponding Author(s): Carmella Evans-Molina, MD, PhD ([email protected]) Indiana University School of Medicine, 635 Barnhill Drive, MS 2031A, Indianapolis, IN 46202, Telephone: (317) 274-4145, Fax (317) 274-4107 Running Title: Golgi Stress Response in Diabetes Word Count: 4358 Number of Figures: 6 Keywords: Golgi apparatus stress, Islets, β cell, Type 1 diabetes, Type 2 diabetes 1 Diabetes Publish Ahead of Print, published online August 20, 2020 Diabetes Page 2 of 781 ABSTRACT The Golgi apparatus (GA) is an important site of insulin processing and granule maturation, but whether GA organelle dysfunction and GA stress are present in the diabetic β-cell has not been tested. We utilized an informatics-based approach to develop a transcriptional signature of β-cell GA stress using existing RNA sequencing and microarray datasets generated using human islets from donors with diabetes and islets where type 1(T1D) and type 2 diabetes (T2D) had been modeled ex vivo. To narrow our results to GA-specific genes, we applied a filter set of 1,030 genes accepted as GA associated. -
Cellular and Molecular Signatures in the Disease Tissue of Early
Cellular and Molecular Signatures in the Disease Tissue of Early Rheumatoid Arthritis Stratify Clinical Response to csDMARD-Therapy and Predict Radiographic Progression Frances Humby1,* Myles Lewis1,* Nandhini Ramamoorthi2, Jason Hackney3, Michael Barnes1, Michele Bombardieri1, Francesca Setiadi2, Stephen Kelly1, Fabiola Bene1, Maria di Cicco1, Sudeh Riahi1, Vidalba Rocher-Ros1, Nora Ng1, Ilias Lazorou1, Rebecca E. Hands1, Desiree van der Heijde4, Robert Landewé5, Annette van der Helm-van Mil4, Alberto Cauli6, Iain B. McInnes7, Christopher D. Buckley8, Ernest Choy9, Peter Taylor10, Michael J. Townsend2 & Costantino Pitzalis1 1Centre for Experimental Medicine and Rheumatology, William Harvey Research Institute, Barts and The London School of Medicine and Dentistry, Queen Mary University of London, Charterhouse Square, London EC1M 6BQ, UK. Departments of 2Biomarker Discovery OMNI, 3Bioinformatics and Computational Biology, Genentech Research and Early Development, South San Francisco, California 94080 USA 4Department of Rheumatology, Leiden University Medical Center, The Netherlands 5Department of Clinical Immunology & Rheumatology, Amsterdam Rheumatology & Immunology Center, Amsterdam, The Netherlands 6Rheumatology Unit, Department of Medical Sciences, Policlinico of the University of Cagliari, Cagliari, Italy 7Institute of Infection, Immunity and Inflammation, University of Glasgow, Glasgow G12 8TA, UK 8Rheumatology Research Group, Institute of Inflammation and Ageing (IIA), University of Birmingham, Birmingham B15 2WB, UK 9Institute of -
Evolution of Vertebrate Solute Carrier Family 9B
etics & E en vo g lu t lo i y o h n a P r f y Journal of Phylogenetics & Holmes et al., J Phylogen Evolution Biol 2016, 4:3 o B l i a o n l r o DOI: 10.4172/2329-9002.1000167 u g o y J Evolutionary Biology ISSN: 2329-9002 Research Article Open Access Evolution of Vertebrate Solute Carrier Family 9B Genes and Proteins (SLC9B): Evidence for a Marsupial Origin for Testis Specific SLC9B1 from an Ancestral Vertebrate SLC9B2 Gene Roger S Holmes1*,2, Kimberly D Spradling-Reeves2 and Laura A Cox2 1Eskitis Institute for Drug Discovery and School of Natural Sciences, Griffith University, Nathan, QLD, Australia 2Department of Genetics and Southwest National Primate Research Center, Texas Biomedical Research Institute, San Antonio, Texas, USA Abstract SLC9B genes and proteins are members of the sodium/lithium hydrogen antiporter family which function as solute exchangers within cellular membranes of mammalian tissues. SLC9B2 and SLC9B1 amino acid sequences and structures and SLC9B-like gene locations were examined using bioinformatic data from several vertebrate genome projects. Vertebrate SLC9B2 sequences shared 56-98% identity as compared with ~50% identities with mammalian SLC9B1 sequences. Sequence alignments, key amino acid residues and conserved predicted transmembrane structures were also studied. Mammalian SLC9B2 and SLC9B1 genes usually contained 11 or 12 coding exons with differential tissue expression patterns: SLC9B2, broad tissue distribution; and SLC9B1, being testis specific. Transcription factor binding sites and CpG islands within the human SLC9B2 and SLC9B1 gene promoters were identified. Phylogenetic analyses suggested thatSLC9B1 originated in an ancestral marsupial genome from a SLC9B2 gene duplication event. -
Novelty Indicator for Enhanced Prioritization of Predicted Gene Ontology Annotations
IEEE/ACM TRANSACTIONS ON COMPUTATIONAL BIOLOGY AND BIOINFORMATICS, VOL. X, NO. X, MONTHXXX 20XX 1 Novelty Indicator for Enhanced Prioritization of Predicted Gene Ontology Annotations Davide Chicco, Fernando Palluzzi, and Marco Masseroli Abstract—Biomolecular controlled annotations have become pivotal in computational biology, because they allow scientists to analyze large amounts of biological data to better understand their test results, and to infer new knowledge. Yet, biomolecular annotation databases are incomplete by definition, like our knowledge of biology, and may contain errors and inconsistent information. In this context, machine-learning algorithms able to predict and prioritize new biomolecular annotations are both effective and efficient, especially if compared with the time-consuming trials of biological validation. To limit the possibility that these techniques predict obvious and trivial high-level features, and to help prioritizing their results, we introduce here a new element that can improve the accuracy and relevance of the results of an annotation prediction and prioritization pipeline. We propose a novelty indicator able to state the level of ”newness” (or ”originality”) of the annotations predicted for a specific gene to Gene Ontology terms, and to help prioritizing the most novel and interesting annotations predicted. We performed a thorough biological functional analysis of the prioritized annotations predicted with high accuracy by using this indicator and our previously proposed prediction algorithms. The relevance -
Shear Stress Modulates Gene Expression in Normal Human Dermal Fibroblasts
University of Calgary PRISM: University of Calgary's Digital Repository Graduate Studies The Vault: Electronic Theses and Dissertations 2017 Shear Stress Modulates Gene Expression in Normal Human Dermal Fibroblasts Zabinyakov, Nikita Zabinyakov, N. (2017). Shear Stress Modulates Gene Expression in Normal Human Dermal Fibroblasts (Unpublished master's thesis). University of Calgary, Calgary, AB. doi:10.11575/PRISM/27775 http://hdl.handle.net/11023/3639 master thesis University of Calgary graduate students retain copyright ownership and moral rights for their thesis. You may use this material in any way that is permitted by the Copyright Act or through licensing that has been assigned to the document. For uses that are not allowable under copyright legislation or licensing, you are required to seek permission. Downloaded from PRISM: https://prism.ucalgary.ca UNIVERSITY OF CALGARY Shear Stress Modulates Gene Expression in Normal Human Dermal Fibroblasts by Nikita Zabinyakov A THESIS SUBMITTED TO THE FACULTY OF GRADUATE STUDIES IN PARTIAL FULFILMENT OF THE REQUIREMENTS FOR THE DEGREE OF MASTER OF SCIENCE GRADUATE PROGRAM IN BIOMEDICAL ENGINEERING CALGARY, ALBERTA JANUARY 2017 © Nikita Zabinyakov 2017 Abstract Applied mechanical forces, such as those resulting from fluid flow, trigger cells to change their functional behavior or phenotype. However, there is little known about how fluid flow affects fibroblasts. The hypothesis of this thesis is that dermal fibroblasts undergo significant changes of expression of differentiation genes after exposure to fluid flow (or shear stress). To test the hypothesis, human dermal fibroblasts were exposed to laminar steady fluid flow for 20 and 40 hours and RNA was collected for microarray analysis. -
Supplementary Material Computational Prediction of SARS
Supplementary_Material Computational prediction of SARS-CoV-2 encoded miRNAs and their putative host targets Sheet_1 List of potential stem-loop structures in SARS-CoV-2 genome as predicted by VMir. Rank Name Start Apex Size Score Window Count (Absolute) Direct Orientation 1 MD13 2801 2864 125 243.8 61 2 MD62 11234 11286 101 211.4 49 4 MD136 27666 27721 104 205.6 119 5 MD108 21131 21184 110 204.7 210 9 MD132 26743 26801 119 188.9 252 19 MD56 9797 9858 128 179.1 59 26 MD139 28196 28233 72 170.4 133 28 MD16 2934 2974 76 169.9 71 43 MD103 20002 20042 80 159.3 403 46 MD6 1489 1531 86 156.7 171 51 MD17 2981 3047 131 152.8 38 87 MD4 651 692 75 140.3 46 95 MD7 1810 1872 121 137.4 58 116 MD140 28217 28252 72 133.8 62 122 MD55 9712 9758 96 132.5 49 135 MD70 13171 13219 93 130.2 131 164 MD95 18782 18820 79 124.7 184 173 MD121 24086 24135 99 123.1 45 176 MD96 19046 19086 75 123.1 179 196 MD19 3197 3236 76 120.4 49 200 MD86 17048 17083 73 119.8 428 223 MD75 14534 14600 137 117 51 228 MD50 8824 8870 94 115.8 79 234 MD129 25598 25642 89 115.6 354 Reverse Orientation 6 MR61 19088 19132 88 197.8 271 10 MR72 23563 23636 148 188.8 286 11 MR11 3775 3844 136 185.1 116 12 MR94 29532 29582 94 184.6 271 15 MR43 14973 15028 109 183.9 226 27 MR14 4160 4206 89 170 241 34 MR35 11734 11792 111 164.2 37 52 MR5 1603 1652 89 152.7 118 53 MR57 18089 18132 101 152.7 139 94 MR8 2804 2864 122 137.4 38 107 MR58 18474 18508 72 134.9 237 117 MR16 4506 4540 72 133.8 311 120 MR34 10010 10048 82 132.7 245 133 MR7 2534 2578 90 130.4 75 146 MR79 24766 24808 75 127.9 59 150 MR65 21528 21576 99 127.4 83 180 MR60 19016 19049 70 122.5 72 187 MR51 16450 16482 75 121 363 190 MR80 25687 25734 96 120.6 75 198 MR64 21507 21544 70 120.3 35 206 MR41 14500 14542 84 119.2 94 218 MR84 26840 26894 108 117.6 94 Sheet_2 List of stable stem-loop structures based on MFE. -
(Q36.1;Q24) with a Concurrent Submicroscopic Del(4)(Q23q24) in an Adult with Polycythemia Vera
cancers Case Report A Novel Acquired t(2;4)(q36.1;q24) with a Concurrent Submicroscopic del(4)(q23q24) in An Adult with Polycythemia Vera Eigil Kjeldsen Cancer Cytogenetic Section, HemoDiagnostic Laboratory, Department of Hematology, Aarhus University Hospital, Tage-Hansens Gade 2, DK-8000 Aarhus C, Denmark; [email protected]; Tel.: +45-7846-7398; Fax: +45-7846-7399 Received: 6 June 2018; Accepted: 21 June 2018; Published: 25 June 2018 Abstract: Background: Polycythemia vera (PV) is a clonal myeloid stem cell disease characterized by a growth-factor independent erythroid proliferation with an inherent tendency to transform into overt acute myeloid malignancy. Approximately 95% of the PV patients harbor the JAK2V617F mutation while less than 35% of the patients harbor cytogenetic abnormalities at the time of diagnosis. Methods and Results: Here we present a JAK2V617F positive PV patient where G-banding revealed an apparently balanced t(2;4)(q35;q21), which was confirmed by 24-color karyotyping. Oligonucleotide array-based Comparative Genomic Hybridization (aCGH) analysis revealed an interstitial 5.4 Mb large deletion at 4q23q24. Locus-specific fluorescent in situ hybridization (FISH) analyses confirmed the mono-allelic 4q deletion and that it was located on der(4)t(2;4). Additional locus-specific bacterial artificial chromosome (BAC) probes and mBanding refined the breakpoint on chromosome 2. With these methods the karyotype was revised to 46,XX,t(2;4)(q36.1;q24)[18]/46,XX[7]. Conclusions: This is the first report on a PV patient associated with an acquired novel t(2;4)(q36.1;q24) and a concurrent submicroscopic deletion del(4)(q23q24). -
Chapter 8 Exome Sequencing in Patient- Parent Trios Reveals New Candidate Genes for Early-Onset Primary Sclerosing Cholangitis
University of Groningen Towards personalized medicine in pediatric inflammatory bowel disease Haisma, Sjoukje DOI: 10.33612/diss.96888808 IMPORTANT NOTE: You are advised to consult the publisher's version (publisher's PDF) if you wish to cite from it. Please check the document version below. Document Version Publisher's PDF, also known as Version of record Publication date: 2019 Link to publication in University of Groningen/UMCG research database Citation for published version (APA): Haisma, S. (2019). Towards personalized medicine in pediatric inflammatory bowel disease. Rijksuniversiteit Groningen. https://doi.org/10.33612/diss.96888808 Copyright Other than for strictly personal use, it is not permitted to download or to forward/distribute the text or part of it without the consent of the author(s) and/or copyright holder(s), unless the work is under an open content license (like Creative Commons). The publication may also be distributed here under the terms of Article 25fa of the Dutch Copyright Act, indicated by the “Taverne” license. More information can be found on the University of Groningen website: https://www.rug.nl/library/open-access/self-archiving-pure/taverne- amendment. Take-down policy If you believe that this document breaches copyright please contact us providing details, and we will remove access to the work immediately and investigate your claim. Downloaded from the University of Groningen/UMCG research database (Pure): http://www.rug.nl/research/portal. For technical reasons the number of authors shown on this cover -
Entrez ID Gene Name Fold Change Q-Value Description
Entrez ID gene name fold change q-value description 4283 CXCL9 -7.25 5.28E-05 chemokine (C-X-C motif) ligand 9 3627 CXCL10 -6.88 6.58E-05 chemokine (C-X-C motif) ligand 10 6373 CXCL11 -5.65 3.69E-04 chemokine (C-X-C motif) ligand 11 405753 DUOXA2 -3.97 3.05E-06 dual oxidase maturation factor 2 4843 NOS2 -3.62 5.43E-03 nitric oxide synthase 2, inducible 50506 DUOX2 -3.24 5.01E-06 dual oxidase 2 6355 CCL8 -3.07 3.67E-03 chemokine (C-C motif) ligand 8 10964 IFI44L -3.06 4.43E-04 interferon-induced protein 44-like 115362 GBP5 -2.94 6.83E-04 guanylate binding protein 5 3620 IDO1 -2.91 5.65E-06 indoleamine 2,3-dioxygenase 1 8519 IFITM1 -2.67 5.65E-06 interferon induced transmembrane protein 1 3433 IFIT2 -2.61 2.28E-03 interferon-induced protein with tetratricopeptide repeats 2 54898 ELOVL2 -2.61 4.38E-07 ELOVL fatty acid elongase 2 2892 GRIA3 -2.60 3.06E-05 glutamate receptor, ionotropic, AMPA 3 6376 CX3CL1 -2.57 4.43E-04 chemokine (C-X3-C motif) ligand 1 7098 TLR3 -2.55 5.76E-06 toll-like receptor 3 79689 STEAP4 -2.50 8.35E-05 STEAP family member 4 3434 IFIT1 -2.48 2.64E-03 interferon-induced protein with tetratricopeptide repeats 1 4321 MMP12 -2.45 2.30E-04 matrix metallopeptidase 12 (macrophage elastase) 10826 FAXDC2 -2.42 5.01E-06 fatty acid hydroxylase domain containing 2 8626 TP63 -2.41 2.02E-05 tumor protein p63 64577 ALDH8A1 -2.41 6.05E-06 aldehyde dehydrogenase 8 family, member A1 8740 TNFSF14 -2.40 6.35E-05 tumor necrosis factor (ligand) superfamily, member 14 10417 SPON2 -2.39 2.46E-06 spondin 2, extracellular matrix protein 3437 -
Investigating the Effect of Chronic Activation of AMP-Activated Protein
Investigating the effect of chronic activation of AMP-activated protein kinase in vivo Alice Pollard CASE Studentship Award A thesis submitted to Imperial College London for the degree of Doctor of Philosophy September 2017 Cellular Stress Group Medical Research Council London Institute of Medical Sciences Imperial College London 1 Declaration I declare that the work presented in this thesis is my own, and that where information has been derived from the published or unpublished work of others it has been acknowledged in the text and in the list of references. This work has not been submitted to any other university or institute of tertiary education in any form. Alice Pollard The copyright of this thesis rests with the author and is made available under a Creative Commons Attribution Non-Commercial No Derivatives license. Researchers are free to copy, distribute or transmit the thesis on the condition that they attribute it, that they do not use it for commercial purposes and that they do not alter, transform or build upon it. For any reuse or redistribution, researchers must make clear to others the license terms of this work. 2 Abstract The prevalence of obesity and associated diseases has increased significantly in the last decade, and is now a major public health concern. It is a significant risk factor for many diseases, including cardiovascular disease (CVD) and type 2 diabetes. Characterised by excess lipid accumulation in the white adipose tissue, which drives many associated pathologies, obesity is caused by chronic, whole-organism energy imbalance; when caloric intake exceeds energy expenditure. Whilst lifestyle changes remain the most effective treatment for obesity and the associated metabolic syndrome, incidence continues to rise, particularly amongst children, placing significant strain on healthcare systems, as well as financial burden. -
Most Chromatin Interactions Are Not in Linkage Disequilibrium
Downloaded from genome.cshlp.org on September 25, 2021 - Published by Cold Spring Harbor Laboratory Press Research Most chromatin interactions are not in linkage disequilibrium Sean Whalen1 and Katherine S. Pollard1,2,3 1Gladstone Institutes, San Francisco, California 94158, USA; 2Department of Epidemiology and Biostatistics, Institute for Human Genetics, Quantitative Biology Institute, and Institute for Computational Health Sciences, University of California San Francisco, San Francisco, California 94158, USA; 3Chan-Zuckerberg Biohub, San Francisco, California 94158, USA Chromatin interactions and linkage disequilibrium (LD) are both pairwise measurements between genomic loci that show block patterns along mammalian chromosomes. Their values are generally high for sites that are nearby in the linear ge- nome but abruptly drop across block boundaries. One function of chromatin boundaries is to insulate regulatory domains from one another. Since recombination is depressed within genes and between distal regulatory elements and their promot- ers, we hypothesized that LD and chromatin contact frequency might be correlated genome-wide with the boundaries of LD blocks and chromatin domains frequently coinciding. To comprehensively address this question, we compared chromatin contacts in 22 cell types to LD across billions of pairs of loci in the human genome. These computationally intensive analyses revealed that there is no concordance between LD and chromatin interactions, even at genomic distances below 25 kilobases (kb) where both tend to be high. At genomic distances where LD is approximately zero, chromatin interactions are frequent. While LD is somewhat elevated between distal regulatory elements and their promoters, LD block boundaries are depleted —not enriched—at chromatin boundaries. Finally, gene expression and ontology data suggest that chromatin contacts identify regulatory variants more reliably than do LD and genomic proximity. -
Most Chromatin Interactions Are Not in Linkage Disequilibrium
Downloaded from genome.cshlp.org on October 5, 2021 - Published by Cold Spring Harbor Laboratory Press Most chromatin interactions are not in linkage disequilibrium Sean Whalen1 and Katherine S. Pollard∗1,2,3 1Gladstone Institutes, San Francisco, CA 94158, USA 2Department of Epidemiology and Biostatistics, Institute for Human Genetics, Quantitative Biology Institute, and Institute for Computational Health Sciences, University of California San Francisco, San Francisco, CA, USA 3Chan-Zuckerberg Biohub, San Francisco, CA, USA December 4, 2018 1 Downloaded from genome.cshlp.org on October 5, 2021 - Published by Cold Spring Harbor Laboratory Press Abstract Chromatin interactions and linkage disequilibrium (LD) are both pairwise measurements between genomic loci that show block patterns along mammalian chromosomes. Their values are generally high for sites that are nearby in the linear genome but abruptly drop across block boundaries. One function of chromatin boundaries is to insulate regulatory domains from one another. Since recombination is depressed within genes and between distal regulatory elements and their promoters, we hypothesized that LD and chromatin contact frequency might be correlated genome-wide with the boundaries of LD blocks and chromatin domains frequently coinciding. To comprehensively address this question, we compared chromatin contacts in 22 cell types to LD across billions of pairs of loci in the human genome. These computationally intensive analyses revealed that there is no concordance between LD and chromatin interactions, even at genomic distances below 25 kilobases where both tend to be high. At genomic distances where LD is approximately zero, chromatin interactions are frequent. While LD is somewhat elevated between distal regulatory elements and their promoters, LD block boundaries are depleted|not enriched|at chromatin boundaries.