A Comparison with Ball Lightning
Total Page:16
File Type:pdf, Size:1020Kb
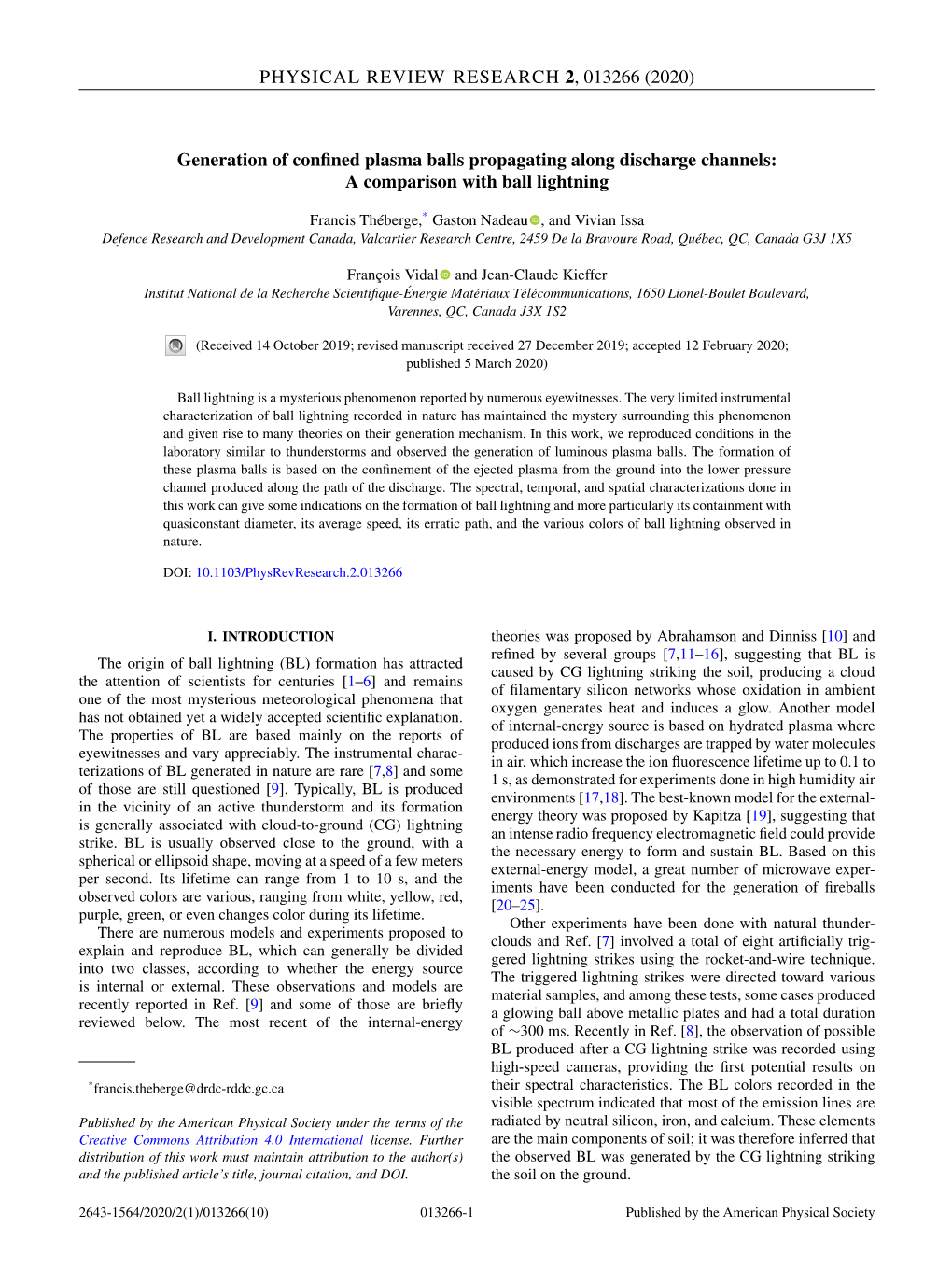
Load more
Recommended publications
-
The Ball Lightning Conundrum
most famous deadis due to ball lightning occun-ed in 1752 or 1753, when die Swedish sci entist Professor Georg Wilhelm Richman was The Ball attempting to repeat Benjamin Franklin's observa dons with a lightning rcxl. An eyewitness report ed that when Richman w;is "a foot away from the iron rod, the] looked at die elecuical indicator Lightning again; just then a palish blue ball of fire, ;LS big as a fist, came out of die rod without any contaa whatst:)ever. It went right to the forehead of die Conundrum professor , who in diat instant fell back without uttering a sound."' Somedmes a luminous globe is said to rapidly descend 6own die path of a linear lightning WILLIAM D STANSFIELD strike and stop near the ground at die impact site. It may dien hover motionless in mid-air or THE EXISTENCE OF BALL UGHTNING HAS move randomly, but most often horizontally, at been questioned for hundreds of years. Today, die relatively slow velocities of walking speed. the phenomenon is a realit>' accepted by most Sometimes it touches or bounces along or near scientists, but how it is foniied and maintiiined the ground, or travels inside buildings, along has yet to be tully explained. Uncritical observers walls, or over floors before being extingui.shed. of a wide variety of glowing atmospheric entities Some balls have been observed to travel along may be prone to call tliem bail lightning. Open- power lines or fences. Wind does not .seem to minded skepdas might wish to delay judgment have any influence on how diese balls move. -
THE ELECTRIC DISCHARGE EXPERIMENTS a Theorist’S Exploration of First Laboratory Plasmas I
THE ELECTRIC DISCHARGE EXPERIMENTS A Theorist’s Exploration of First Laboratory Plasmas I. INTRODUCTION • Who? • Experimentalists interested in electrical properties of matter • What? • Experiments with electromagnetic fields in low-pressure Gases • Where? • Germany and Britain • When? • Late nineteenth / Early twentieth centuries • Why? • Unify classical theories for light and matter • kinetic theory, electromagnetic theory • “The study of the electrical properties of gases seems to offer the most promising field for investigatinG the Nature of Electricity and Matter, for thanks to the Kinetic Theory of Gases our idea of non-electric processes in gases is much more vivid than they are for liquids or solids”– JJ Thomson [Thomson, 1896] II. RESULTS • Failure to unify classical theories for light and matter • Evidence of complex electromagnetic interactions between light and matter at the sub-atomic level • Experimental basis for Modern Era of Physics • "a New Era has beGun in Physics, in which the electrical properties of gases have played and will play a most important part.“ JJ Thomson [Thomson, 1896] III. DISCHARGE TUBE • DischarGe Tube • Weakly-Conducting Exterior Solid • Weakly-Conducting Interior Gas (WCG) • Strongly-Conducting Interior Gas (SCG) • Applied Interior Pressure (P) • Electrodes (Cathode and Anode) • Model as Ideal Capacitor with Dielectric (D) • Applied Potential Difference (V) • Applied Electric Field (E) • Particle Drifts • Electric field transfers energy to charged particles • Particles scatter in the Gas interior -
Current-Voltage Characteristics of DC Plasma Discharges Employed In
IRAQI JOURNAL OF APPLIED PHYSICS Current-Voltage Mohammed K. Khalaf 1 Characteristics of DC Plasma Oday A. Hammadi 2 Firas J. Kadhim 3 Discharges Employed in Sputtering Techniques In this work, the current-voltage characteristics of dc plasma discharges were 1 Ministry of Science studied. The current-voltage characteristics of argon gas discharge at different and Technology, inter-electrode distances and working pressure of 0.7mbar without magnetrons Baghdad, IRAQ 2 were introduced. Then the variation of discharge current with inter-electrode Department of Physics, distance at certain discharge voltages without using magnetron was determined. College of Education, The current-voltage characteristics of discharge plasma at inter-electrode Al-Iraqia University, Baghdad, IRAQ distance of 4cm without magnetron, with only one magnetron and with dual 3 Department of Physics, magnetrons were also determined. The variation of discharge current with inter- College of Science, electrode distance at certain discharge voltage (400V) for the cases without University of Baghdad, magnetron, using one magnetron and dual magnetrons were studied. Finally, the Baghdad, IRAQ discharge current-voltage characteristics for different argon/nitrogen mixtures at total gas pressure of 0.7mbar and inter-electrode distance of 4cm were presented. Keywords: Plasma discharge; Magnetron; Glow discharge; Sputtering 1. Introduction emission effects, which lead to increase the flowing Glow discharge is low-temperature neutral current gradually approaching the breakdown point plasma where the number of electrons is equal to the beyond which the avalanche occurs and the current number of ions despite that local but negligible increases drastically in the Townsend discharge imbalances may exist at walls [1]. -
Plasma Discharge in Water and Its Application for Industrial Cooling Water Treatment
Plasma Discharge in Water and Its Application for Industrial Cooling Water Treatment A Thesis Submitted to the Faculty of Drexel University by Yong Yang In partial fulfillment of the Requirements for the degree of Doctor of Philosophy June 2011 ii © Copyright 2008 Yong Yang. All Rights Reserved. iii Acknowledgements I would like to express my greatest gratitude to both my advisers Prof. Young I. Cho and Prof. Alexander Fridman. Their help, support and guidance were appreciated throughout my graduate studies. Their experience and expertise made my five year at Drexel successful and enjoyable. I would like to convey my deep appreciation to the most dedicated Dr. Alexander Gutsol and Dr. Andrey Starikovskiy, with whom I had pleasure to work with on all these projects. I feel thankful for allowing me to walk into their office any time, even during their busiest hours, and I’m always amazed at the width and depth of their knowledge in plasma physics. Also I would like to thank Profs. Ying Sun, Gary Friedman, and Alexander Rabinovich for their valuable advice on this thesis as committee members. I am thankful for the financial support that I received during my graduate study, especially from the DOE grants DE-FC26-06NT42724 and DE-NT0005308, the Drexel Dean’s Fellowship, George Hill Fellowship, and the support from the Department of Mechanical Engineering and Mechanics. I would like to thank the friendship and help from the friends and colleagues at Drexel Plasma Institute over the years. Special thanks to Hyoungsup Kim and Jin Mu Jung. Without their help I would not be able to finish the fouling experiments alone. -
Arxiv:1303.1588V2 [Physics.Hist-Ph] 8 Jun 2015 4.4 Errors in the Article
Translation of an article by Paul Drude in 1904 Translation by A. J. Sederberg1;∗ (pp. 512{519, 560{561), J. Burkhart2;y (pp. 519{527), and F. Apfelbeck3;z (pp. 528{560) Discussion by B. H. McGuyer1;x 1Department of Physics, Princeton University, Princeton, New Jersey 08544, USA 2Department of Physics, Columbia University, 538 West 120th Street, New York, NY 10027-5255, USA June 9, 2015 Abstract English translation of P. Drude, Annalen der Physik 13, 512 (1904), an article by Paul Drude about Tesla transformers and wireless telegraphy. Includes a discussion of the derivation of an equivalent circuit and the prediction of nonreciprocal mutual inductance for Tesla transformers in the article, which is supplementary material for B. McGuyer, PLoS ONE 9, e115397 (2014). Contents 1 Introduction 2 2 Bibliographic information 2 3 Translation 3 I. Definition and Integration of the Differential Equations . .3 Figure 1 . .5 II. The Magnetic Coupling is Very Small . .9 III. Measurement of the Period and the Damping . 12 IV. The Magnetic Coupling is Not Very Small . 19 VII. Application to Wireless Telegraphy . 31 Figure 2 . 32 Figure 3 . 33 Summary of Main Results . 39 4 Discussion 41 4.1 Technical glossary . 41 4.2 Hopkinson's law . 41 4.3 Equivalent circuit parameters from the article . 42 arXiv:1303.1588v2 [physics.hist-ph] 8 Jun 2015 4.4 Errors in the article . 42 4.5 Modifications to match Ls with previous work . 42 ∗Present address: Division of Biological Sciences, University of Chicago, 5812 South Ellis Avenue, Chicago, IL 60637, USA. yPresent address: Department of Microsystems and Informatics, Hochschule Kaiserslautern, Amerikastrasse 1, D-66482 Zweibr¨ucken, Germany. -
The Modelling and Characterization of Dielectric Barrier Discharge-Based Cold Plasma Jets
The Modelling and Characterization of Dielectric Barrier Discharge-Based Cold Plasma Jets The Modelling and Characterization of Dielectric Barrier Discharge-Based Cold Plasma Jets By G. Divya Deepak, Narendra Kumar Joshi and Ram Prakash The Modelling and Characterization of Dielectric Barrier Discharge-Based Cold Plasma Jets By G. Divya Deepak, Narendra Kumar Joshi and Ram Prakash This book first published 2020 Cambridge Scholars Publishing Lady Stephenson Library, Newcastle upon Tyne, NE6 2PA, UK British Library Cataloguing in Publication Data A catalogue record for this book is available from the British Library Copyright © 2020 by G. Divya Deepak, Narendra Kumar Joshi and Ram Prakash All rights for this book reserved. No part of this book may be reproduced, stored in a retrieval system, or transmitted, in any form or by any means, electronic, mechanical, photocopying, recording or otherwise, without the prior permission of the copyright owner. ISBN (10): 1-5275-4539-3 ISBN (13): 978-1-5275-4539-7 CONTENTS List of Figures........................................................................................... viii List of Tables ............................................................................................. xii List of Abbreviations ................................................................................ xiii Acknowledgements ................................................................................... xv Preface ..................................................................................................... -
Plasma of Underwater Electric Discharges with Metal Vapors V.F
PLASMA OF UNDERWATER ELECTRIC DISCHARGES WITH METAL VAPORS V.F. Boretskij1, A.N. Veklich1, T.A. Tmenova1, Y. Cressault2, F. Valensi2, K.G. Lopatko3, Y.G. Aftandilyants3 1Taras Shevchenko National University of Kyiv, Kyiv, Ukraine; 2Universite Paul Sabatier, Toulouse, France; 3National University of Life and Environmental Sciences of Ukraine, Kyiv, Ukraine E-mail: [email protected]; [email protected]; [email protected] This paper deals with spectroscopy of underwater electric discharge plasma with. In particular, the focus is on configuration where the electrodes are immersed in liquid and its application in nanoscience and biotechnology. General overview of the experimental approach adopted by authors aiming to study the water-submerged electrical discharge plasma and effects of various parameters on its properties is described. The electron density was estimated on the base of spectral line broadening and shifting. PACS: 52.25.−b, 52.80.−s, 52.80.Wq INTRODUCTION components are typically studied in distinct fields of PLASMAS IN LIQUID research. Plasmas in liquid are becoming an increasingly PLASMA-LIQUID INTERACTIONS FOR important topic in the field of plasma science and NANOMATERIAL SYNTHESIS technology. In the last two decades, attention of research on the The focus of this paper, in particular, is directed interactions of plasmas with liquids has spread on a towards a relatively new branch of plasma research, variety of applications that include electrical switching nanomaterial synthesis through plasma–liquid [1], analytical chemistry [2], environmental remediation interactions, which has been developing rapidly, mainly [3, 4], sterilization and medical applications [5], etc. due to the various recently developed plasma sources These opportunities have challenged plasma community operating at low and atmospheric pressures. -
Static Electric Discharge Hazard on Bulk Oil Tank Vessels
Static Electric Discharge Hazard On Bulk Oil Tank Vessels Phase 1 Report Prepared for: Commandant G-MTH-2, Engineering Branch US Coast Guard Headquarters 2100 2nd Street, SW Washington, DC 20593-0001 Prepared by: Michael G. Dyer, DTS-73 The Volpe National Transportation Systems Center 55 Broadway, Kendall Square Cambridge, Massachusetts 02142-1093 For more information on this report contact: Guy Collona and Bob Benedetti National Fire Protection Association Batterymarch Park Quincy, Massachusetts 02269 Michael Dyer US Department of Transportation Research and Special Programs Administration John A. Volpe National Transportation Systems Center Kendall Square Cambridge, Massachusetts 02142 1 Table of Contents 1. Executive Summary 2. Introduction and Background .1 The problem .2 Accident history .3 Safety measures in place .4 Project goals 3. Recent Accident History .1 FIONA .2 AMERICAN EAGLE .3 Tank barge TT 103 .4 Tank barge STC 410 .5 Tank barge Hollywood 1034 .6 Other accidents .7 Overview 4. The Electrostatic Hazard .1 Four conditions required for explosive ignition .2 Mechanisms for producing hazardous conditions .1 Static generation .2 Accumulation of charge and potential .3 Spark discharge .4 Flammable vapor 5. Corrective and Preventative Measures .1 Mitigation of static generation .1 Loading precautions .2 Displacing of lines .3 Precaution against mist and steam .4 Precaution for crude oil washing (COW .5 Precaution for overall loading .6 Air injection precaution .7 Precaution for combination carriers .2 Prevention of charge accumulation -
Electrostatic Hazards Assessment of Nitramine Explosives: Resistivity, Charge Accumulation and Discharge Sensitivity
Central European Journal of Energetic Materials ISSN 1733-7178; e-ISSN 2353-1843 Copyright © 2016 Institute of Industrial Organic Chemistry, Poland Cent. Eur. J. Energ. Mater., 2016, 13(3), 755-769; DOI: 10.22211/cejem/65009 Electrostatic Hazards Assessment of Nitramine Explosives: Resistivity, Charge Accumulation and Discharge Sensitivity Qiqi PENG,1 Wei CAO,2 Wentao ZHOU,1 Zhongqi HE,1* Wei JIANG,1,3 Wanghua CHEN 1 1 School of Chemical Engineering, Nanjing University of Science and Technology, Nanjing 210094, P.R. China 2 Institute of Chemical Materials, China Academy of Engineering Physics, Mianyang 621900, P.R. China 3 National Special Superfine Powder Engineering Research Center of China, Nanjing 210094, P.R. China *E-mail: [email protected] Abstract: The electrostatic hazards of nitramine explosives (RDX, HMX) were assessed in this paper. The resistivities of different particle-size RDX and HMX were tested by a device designed and manufactured according to the standard ISO/ IEC 80079-20-2:2016. This work shows that the resistivities of uncompacted RDX and HMX increase as the particle size decreases. Charging characteristics test experiments were also carried out using a so-called sieve method. Using this method, the influence of aperture size on charge accumulation of RDX was studied, and the characteristics of electrostatic accumulation of different particle-size RDX and HMX sieved with 50 mesh standard sieve were compared. The results show that the absolute value of the charge accumulation increases as the mesh number increases (i.e. the aperture size decreases), and increases as the particle size is decreased, indicating that nano-sized RDX and nano-sized HMX accumulate static electricity more easily than conventional micron-sized ones. -
IS 1885-1 (1961): Electrotechnical Vocabulary, Part 1: Fundamental Definitions [ETD 1: Basic Electrotechnical Standards]
इंटरनेट मानक Disclosure to Promote the Right To Information Whereas the Parliament of India has set out to provide a practical regime of right to information for citizens to secure access to information under the control of public authorities, in order to promote transparency and accountability in the working of every public authority, and whereas the attached publication of the Bureau of Indian Standards is of particular interest to the public, particularly disadvantaged communities and those engaged in the pursuit of education and knowledge, the attached public safety standard is made available to promote the timely dissemination of this information in an accurate manner to the public. “जान का अधकार, जी का अधकार” “परा को छोड न 5 तरफ” Mazdoor Kisan Shakti Sangathan Jawaharlal Nehru “The Right to Information, The Right to Live” “Step Out From the Old to the New” IS 1885-1 (1961): Electrotechnical vocabulary, Part 1: Fundamental definitions [ETD 1: Basic Electrotechnical Standards] “ान $ एक न भारत का नमण” Satyanarayan Gangaram Pitroda “Invent a New India Using Knowledge” “ान एक ऐसा खजाना > जो कभी चराया नह जा सकताह ै”ै Bhartṛhari—Nītiśatakam “Knowledge is such a treasure which cannot be stolen” IS : 1885 (Part 1) - 1961 (Reaffirmed 1996) Indian Standard ELECTROTECHNICAL VOCABULARY PART I FUNDAMENTAL DEFINITIONS Sixth Reprint NOVEMBER 2000 ( Incorporating Amendments No. 1 and 2 ) UDC 001.4 : 621.3.01 © Copyright 1969 BUREAU OF INDIAN STANDARDS MANAK BHAVAN, 9 BAHADUR SHAH ZAFAR MARG NEW DELHI 110002 Gr 9 JUNE 1962 IS : 1885 (Part I) - 1961 Indian Standard ELECTROTECHNICAL VOCABULARY PART I FUNDAMENTAL DEFINITIONS Electrotechnical Standards Sectional Committee, ETDC 1 Chairman Representing SHRI M. -
Characterization of Electrical Discharge Machining Plasmas
CHARACTERIZATION OF ELECTRICAL DISCHARGE MACHINING PLASMAS THÈSE NO 3542 (2006) PRÉSENTÉE LE 9 JUIN 2006 À LA faculté SCIENCES DE BASE Centre de Recherche en Physique des Plasmas SECTION DE PHYSIQUE ÉCOLE POLYTECHNIQUE FÉDÉRALE DE LAUSANNE POUR L'OBTENTION DU GRADE DE DOCTEUR ÈS SCIENCES PAR Antoine DESCOEUDRES ingénieur physicien diplômé EPF de nationalité suisse et originaire de La Sagne (NE) acceptée sur proposition du jury: Prof. R. Schaller, président du jury Dr Ch. Hollenstein, directeur de thèse Prof. M. Rappaz, rapporteur Dr G. Wälder, rapporteur Prof. J. Winter, rapporteur Lausanne, EPFL 2006 “La frousse, moi ! ... J’aime autant vous dire, mille tonnerres ! que quand je le rencontrerai, votre yéti, ça va faire des étincelles !” le capitaine Haddock Abstract Electrical Discharge Machining (EDM) is a well-known machining technique since more than fifty years. Its principle is to use the eroding effect on the electrodes of successive electric spark discharges created in a dielectric liquid. EDM is nowadays widely-used in a large number of industrial areas. Nevertheless, few studies have been done on the discharge itself and on the plasma created during this process. Further improvements of EDM, especially for micro-machining, require a better control and understanding of the discharge and of its interaction with the electrodes. In this work, the different phases of the EDM process and the properties of the EDM plasma have been systematically investigated with electrical measurements, with imaging and with time- and spatially- resolved optical emission spectroscopy. The pre-breakdown phase in water is characterized by the generation of numerous small hydrogen bubbles, created by electrolysis. -
Tesla's High Voltage and High Frequency Generators with Oscillatory Circuits with Each Other the Capacitance Are Great on Primary Side and Small on Secondary
SERBIAN JOURNAL OF ELECTRICAL ENGINEERING Vol. 13, No. 3, October 2016, 301-333 UDC: 621.317.32+621.373 DOI: 10.2298/SJEE1603301C Tesla’s High Voltage and High Frequency Generators with Oscillatory Circuits* Jovan M. Cvetić1 Abstract: The principles that represent the basics of the work of the high voltage and high frequency generator with oscillating circuits will be discussed. Until 1891, Tesla made and used mechanical generators with a large number of extruded poles for the frequencies up to about 20 kHz. The first electric generators based on a new principle of a weakly coupled oscillatory circuits he used for the wireless signal transmission, for the study of the discharges in vacuum tubes, the wireless energy transmission, for the production of the cathode rays, that is x-rays and other experiments. Aiming to transfer the signals and the energy to any point of the surface of the Earth, in the late of 19th century, he had discovered and later patented a new type of high frequency generator called a magnifying transmitter. He used it to examine the propagation of electromagnetic waves over the surface of the Earth in experiments in Colorado Springs in the period 1899-1900. Tesla observed the formation of standing electromagnetic waves on the surface of the Earth by measuring radiated electric field from distant lightning thunderstorm. He got the idea to generate the similar radiation to produce the standing waves. On the one hand, signal transmission, i.e. communication at great distances would be possible and on the other hand, with more powerful and with at least three magnifying transmitters the wireless transmission of energy without conductors at any point of the Earth surface could also be achieved.