Lecture 1 Derived Algebraic Geometry 1. Simplicial Commutative Rings. We
Total Page:16
File Type:pdf, Size:1020Kb
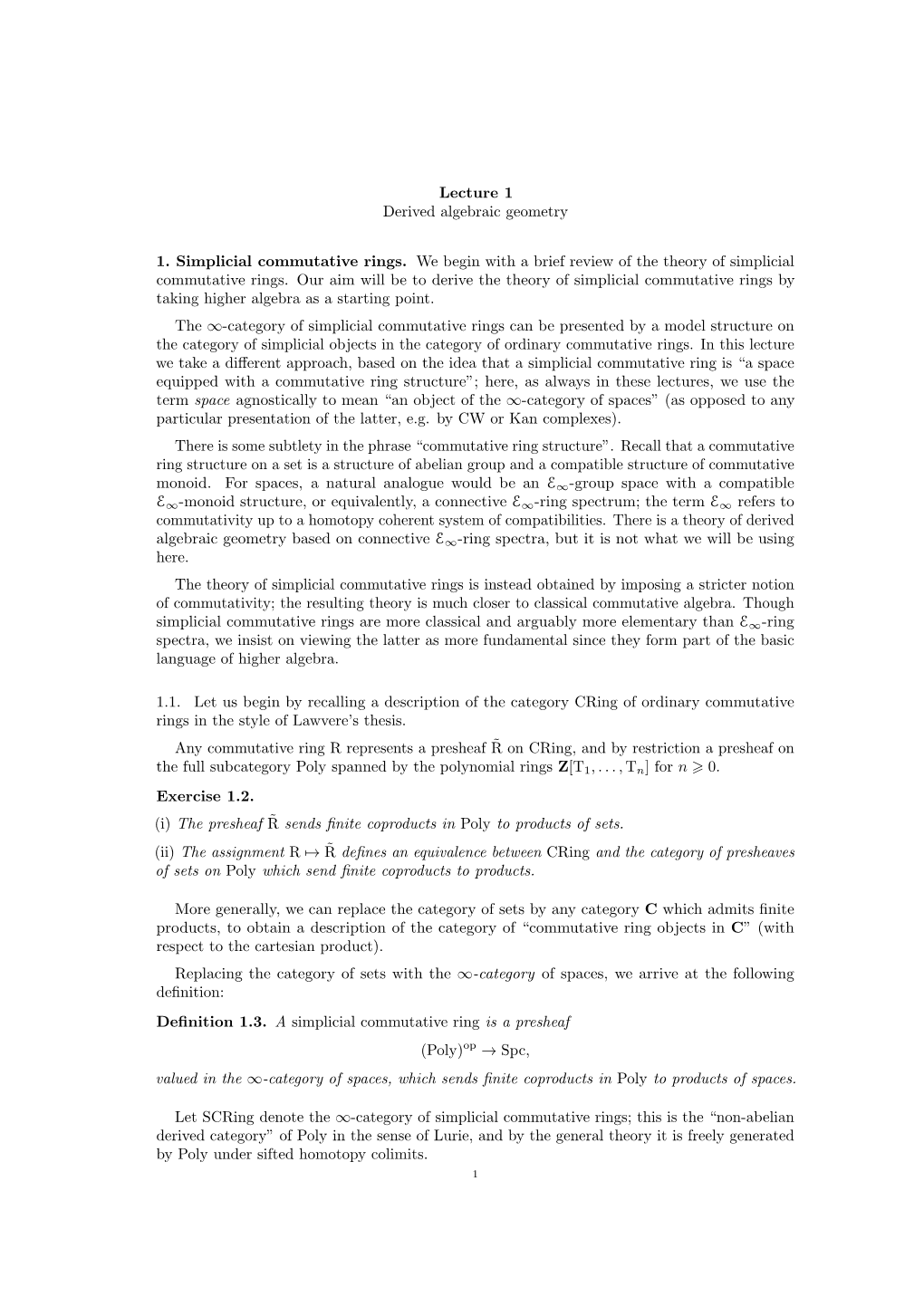
Load more
Recommended publications
-
On the Cohen-Macaulay Modules of Graded Subrings
TRANSACTIONS OF THE AMERICAN MATHEMATICAL SOCIETY Volume 357, Number 2, Pages 735{756 S 0002-9947(04)03562-7 Article electronically published on April 27, 2004 ON THE COHEN-MACAULAY MODULES OF GRADED SUBRINGS DOUGLAS HANES Abstract. We give several characterizations for the linearity property for a maximal Cohen-Macaulay module over a local or graded ring, as well as proofs of existence in some new cases. In particular, we prove that the existence of such modules is preserved when taking Segre products, as well as when passing to Veronese subrings in low dimensions. The former result even yields new results on the existence of finitely generated maximal Cohen-Macaulay modules over non-Cohen-Macaulay rings. 1. Introduction and definitions The notion of a linear maximal Cohen-Macaulay module over a local ring (R; m) was introduced by Ulrich [17], who gave a simple characterization of the Gorenstein property for a Cohen-Macaulay local ring possessing a maximal Cohen-Macaulay module which is sufficiently close to being linear, as defined below. A maximal Cohen-Macaulay module (abbreviated MCM module)overR is one whose depth is equal to the Krull dimension of the ring R. All modules to be considered in this paper are assumed to be finitely generated. The minimal number of generators of an R-module M, which is equal to the (R=m)-vector space dimension of M=mM, will be denoted throughout by ν(M). In general, the length of a finitely generated Artinian module M is denoted by `(M)(orby`R(M), if it is necessary to specify the ring acting on M). -
Modules and Lie Semialgebras Over Semirings with a Negation Map 3
MODULES AND LIE SEMIALGEBRAS OVER SEMIRINGS WITH A NEGATION MAP GUY BLACHAR Abstract. In this article, we present the basic definitions of modules and Lie semialgebras over semirings with a negation map. Our main example of a semiring with a negation map is ELT algebras, and some of the results in this article are formulated and proved only in the ELT theory. When dealing with modules, we focus on linearly independent sets and spanning sets. We define a notion of lifting a module with a negation map, similarly to the tropicalization process, and use it to prove several theorems about semirings with a negation map which possess a lift. In the context of Lie semialgebras over semirings with a negation map, we first give basic definitions, and provide parallel constructions to the classical Lie algebras. We prove an ELT version of Cartan’s criterion for semisimplicity, and provide a counterexample for the naive version of the PBW Theorem. Contents Page 0. Introduction 2 0.1. Semirings with a Negation Map 2 0.2. Modules Over Semirings with a Negation Map 3 0.3. Supertropical Algebras 4 0.4. Exploded Layered Tropical Algebras 4 1. Modules over Semirings with a Negation Map 5 1.1. The Surpassing Relation for Modules 6 1.2. Basic Definitions for Modules 7 1.3. -morphisms 9 1.4. Lifting a Module Over a Semiring with a Negation Map 10 1.5. Linearly Independent Sets 13 1.6. d-bases and s-bases 14 1.7. Free Modules over Semirings with a Negation Map 18 2. -
Irreducible Representations of Finite Monoids
U.U.D.M. Project Report 2019:11 Irreducible representations of finite monoids Christoffer Hindlycke Examensarbete i matematik, 30 hp Handledare: Volodymyr Mazorchuk Examinator: Denis Gaidashev Mars 2019 Department of Mathematics Uppsala University Irreducible representations of finite monoids Christoffer Hindlycke Contents Introduction 2 Theory 3 Finite monoids and their structure . .3 Introductory notions . .3 Cyclic semigroups . .6 Green’s relations . .7 von Neumann regularity . 10 The theory of an idempotent . 11 The five functors Inde, Coinde, Rese,Te and Ne ..................... 11 Idempotents and simple modules . 14 Irreducible representations of a finite monoid . 17 Monoid algebras . 17 Clifford-Munn-Ponizovski˘ıtheory . 20 Application 24 The symmetric inverse monoid . 24 Calculating the irreducible representations of I3 ........................ 25 Appendix: Prerequisite theory 37 Basic definitions . 37 Finite dimensional algebras . 41 Semisimple modules and algebras . 41 Indecomposable modules . 42 An introduction to idempotents . 42 1 Irreducible representations of finite monoids Christoffer Hindlycke Introduction This paper is a literature study of the 2016 book Representation Theory of Finite Monoids by Benjamin Steinberg [3]. As this book contains too much interesting material for a simple master thesis, we have narrowed our attention to chapters 1, 4 and 5. This thesis is divided into three main parts: Theory, Application and Appendix. Within the Theory chapter, we (as the name might suggest) develop the necessary theory to assist with finding irreducible representations of finite monoids. Finite monoids and their structure gives elementary definitions as regards to finite monoids, and expands on the basic theory of their structure. This part corresponds to chapter 1 in [3]. The theory of an idempotent develops just enough theory regarding idempotents to enable us to state a key result, from which the principal result later follows almost immediately. -
Algebraic Number Theory
Algebraic Number Theory William B. Hart Warwick Mathematics Institute Abstract. We give a short introduction to algebraic number theory. Algebraic number theory is the study of extension fields Q(α1; α2; : : : ; αn) of the rational numbers, known as algebraic number fields (sometimes number fields for short), in which each of the adjoined complex numbers αi is algebraic, i.e. the root of a polynomial with rational coefficients. Throughout this set of notes we use the notation Z[α1; α2; : : : ; αn] to denote the ring generated by the values αi. It is the smallest ring containing the integers Z and each of the αi. It can be described as the ring of all polynomial expressions in the αi with integer coefficients, i.e. the ring of all expressions built up from elements of Z and the complex numbers αi by finitely many applications of the arithmetic operations of addition and multiplication. The notation Q(α1; α2; : : : ; αn) denotes the field of all quotients of elements of Z[α1; α2; : : : ; αn] with nonzero denominator, i.e. the field of rational functions in the αi, with rational coefficients. It is the smallest field containing the rational numbers Q and all of the αi. It can be thought of as the field of all expressions built up from elements of Z and the numbers αi by finitely many applications of the arithmetic operations of addition, multiplication and division (excepting of course, divide by zero). 1 Algebraic numbers and integers A number α 2 C is called algebraic if it is the root of a monic polynomial n n−1 n−2 f(x) = x + an−1x + an−2x + ::: + a1x + a0 = 0 with rational coefficients ai. -
A Topology for Operator Modules Over W*-Algebras Bojan Magajna
Journal of Functional AnalysisFU3203 journal of functional analysis 154, 1741 (1998) article no. FU973203 A Topology for Operator Modules over W*-Algebras Bojan Magajna Department of Mathematics, University of Ljubljana, Jadranska 19, Ljubljana 1000, Slovenia E-mail: Bojan.MagajnaÄuni-lj.si Received July 23, 1996; revised February 11, 1997; accepted August 18, 1997 dedicated to professor ivan vidav in honor of his eightieth birthday Given a von Neumann algebra R on a Hilbert space H, the so-called R-topology is introduced into B(H), which is weaker than the norm and stronger than the COREultrastrong operator topology. A right R-submodule X of B(H) is closed in the Metadata, citation and similar papers at core.ac.uk Provided byR Elsevier-topology - Publisher if and Connector only if for each b #B(H) the right ideal, consisting of all a # R such that ba # X, is weak* closed in R. Equivalently, X is closed in the R-topology if and only if for each b #B(H) and each orthogonal family of projections ei in R with the sum 1 the condition bei # X for all i implies that b # X. 1998 Academic Press 1. INTRODUCTION Given a C*-algebra R on a Hilbert space H, a concrete operator right R-module is a subspace X of B(H) (the algebra of all bounded linear operators on H) such that XRX. Such modules can be characterized abstractly as L -matricially normed spaces in the sense of Ruan [21], [11] which are equipped with a completely contractive R-module multi- plication (see [6] and [9]). -
Abstract Algebra: Monoids, Groups, Rings
Notes on Abstract Algebra John Perry University of Southern Mississippi [email protected] http://www.math.usm.edu/perry/ Copyright 2009 John Perry www.math.usm.edu/perry/ Creative Commons Attribution-Noncommercial-Share Alike 3.0 United States You are free: to Share—to copy, distribute and transmit the work • to Remix—to adapt the work Under• the following conditions: Attribution—You must attribute the work in the manner specified by the author or licen- • sor (but not in any way that suggests that they endorse you or your use of the work). Noncommercial—You may not use this work for commercial purposes. • Share Alike—If you alter, transform, or build upon this work, you may distribute the • resulting work only under the same or similar license to this one. With the understanding that: Waiver—Any of the above conditions can be waived if you get permission from the copy- • right holder. Other Rights—In no way are any of the following rights affected by the license: • Your fair dealing or fair use rights; ◦ Apart from the remix rights granted under this license, the author’s moral rights; ◦ Rights other persons may have either in the work itself or in how the work is used, ◦ such as publicity or privacy rights. Notice—For any reuse or distribution, you must make clear to others the license terms of • this work. The best way to do this is with a link to this web page: http://creativecommons.org/licenses/by-nc-sa/3.0/us/legalcode Table of Contents Reference sheet for notation...........................................................iv A few acknowledgements..............................................................vi Preface ...............................................................................vii Overview ...........................................................................vii Three interesting problems ............................................................1 Part . -
On Finite Semifields with a Weak Nucleus and a Designed
On finite semifields with a weak nucleus and a designed automorphism group A. Pi~nera-Nicol´as∗ I.F. R´uay Abstract Finite nonassociative division algebras S (i.e., finite semifields) with a weak nucleus N ⊆ S as defined by D.E. Knuth in [10] (i.e., satisfying the conditions (ab)c − a(bc) = (ac)b − a(cb) = c(ab) − (ca)b = 0, for all a; b 2 N; c 2 S) and a prefixed automorphism group are considered. In particular, a construction of semifields of order 64 with weak nucleus F4 and a cyclic automorphism group C5 is introduced. This construction is extended to obtain sporadic finite semifields of orders 256 and 512. Keywords: Finite Semifield; Projective planes; Automorphism Group AMS classification: 12K10, 51E35 1 Introduction A finite semifield S is a finite nonassociative division algebra. These rings play an important role in the context of finite geometries since they coordinatize projective semifield planes [7]. They also have applications on coding theory [4, 8, 6], combinatorics and graph theory [13]. During the last few years, computational efforts in order to clasify some of these objects have been made. For instance, the classification of semifields with 64 elements is completely known, [16], as well as those with 243 elements, [17]. However, many questions are open yet: there is not a classification of semifields with 128 or 256 elements, despite of the powerful computers available nowadays. The knowledge of the structure of these concrete semifields can inspire new general constructions, as suggested in [9]. In this sense, the work of Lavrauw and Sheekey [11] gives examples of constructions of semifields which are neither twisted fields nor two-dimensional over a nucleus starting from the classification of semifields with 64 elements. -
Modules Over the Steenrod Algebra
View metadata, citation and similar papers at core.ac.uk brought to you by CORE provided by Elsevier - Publisher Connector Tcwhcv’ Vol. 10. PD. 211-282. Pergamon Press, 1971. Printed in Great Britain MODULES OVER THE STEENROD ALGEBRA J. F. ADAMS and H. R. MARGOLIS (Received 4 November 1970 ; revised 26 March 197 1) 90. INTRODUCTION IN THIS paper we will investigate certain aspects of the structure of the mod 2 Steenrod algebra, A, and of modules over it. Because of the central role taken by the cohomology groups of spaces considered as A-modules in much of recent algebraic topology (e.g. [ 11, [2], [7]), it is not unreasonable to suppose that the algebraic structure that we elucidate will be of benefit in the study of topological problems. The main result of this paper, Theorem 3.1, gives a criterion for a module !M to be free over the Steenrod algebra, or over some subHopf algebra B of A. To describe this criterion consider a ring R and a left module over R, M; for e E R we define the map e: M -+ M by e(m) = em. If the element e satisfies the condition that e2 = 0 then im e c ker e, therefore we can define the homology group H(M, e) = ker elime. Then, for example, H(R, e) = 0 implies that H(F, e) = 0 for any free R-module F. We can now describe the criterion referred to above: for any subHopf algebra B of A there are elements e,-with ei2 = 0 and H(B, ei) = O-such that a connected B-module M is free over B if and only if H(M, ei) = 0 for all i. -
Ring (Mathematics) 1 Ring (Mathematics)
Ring (mathematics) 1 Ring (mathematics) In mathematics, a ring is an algebraic structure consisting of a set together with two binary operations usually called addition and multiplication, where the set is an abelian group under addition (called the additive group of the ring) and a monoid under multiplication such that multiplication distributes over addition.a[›] In other words the ring axioms require that addition is commutative, addition and multiplication are associative, multiplication distributes over addition, each element in the set has an additive inverse, and there exists an additive identity. One of the most common examples of a ring is the set of integers endowed with its natural operations of addition and multiplication. Certain variations of the definition of a ring are sometimes employed, and these are outlined later in the article. Polynomials, represented here by curves, form a ring under addition The branch of mathematics that studies rings is known and multiplication. as ring theory. Ring theorists study properties common to both familiar mathematical structures such as integers and polynomials, and to the many less well-known mathematical structures that also satisfy the axioms of ring theory. The ubiquity of rings makes them a central organizing principle of contemporary mathematics.[1] Ring theory may be used to understand fundamental physical laws, such as those underlying special relativity and symmetry phenomena in molecular chemistry. The concept of a ring first arose from attempts to prove Fermat's last theorem, starting with Richard Dedekind in the 1880s. After contributions from other fields, mainly number theory, the ring notion was generalized and firmly established during the 1920s by Emmy Noether and Wolfgang Krull.[2] Modern ring theory—a very active mathematical discipline—studies rings in their own right. -
Derived Differential Geometry
The definition of schemes Different kinds of spaces in algebraic geometry Some basics of category theory What is derived geometry? Moduli spaces and moduli functors Algebraic spaces and (higher) stacks Derived Differential Geometry Lecture 1 of 14: Background material in algebraic geometry and category theory Dominic Joyce, Oxford University Summer 2015 These slides, and references, etc., available at http://people.maths.ox.ac.uk/∼joyce/DDG2015 1 / 48 Dominic Joyce, Oxford University Lecture 1: Algebraic Geometry and Category Theory The definition of schemes Different kinds of spaces in algebraic geometry Some basics of category theory What is derived geometry? Moduli spaces and moduli functors Algebraic spaces and (higher) stacks Plan of talk: 1 Different kinds of spaces in algebraic geometry 1.1 The definition of schemes 1.2 Some basics of category theory 1.3 Moduli spaces and moduli functors 1.4 Algebraic spaces and (higher) stacks 2 / 48 Dominic Joyce, Oxford University Lecture 1: Algebraic Geometry and Category Theory The definition of schemes Different kinds of spaces in algebraic geometry Some basics of category theory What is derived geometry? Moduli spaces and moduli functors Algebraic spaces and (higher) stacks 1. Different kinds of spaces in algebraic geometry Algebraic geometry studies spaces built using algebras of functions. Here are the main classes of spaces studied by algebraic geometers, in order of complexity, and difficulty of definition: Smooth varieties (e.g. Riemann surfaces, or algebraic complex n manifolds such as CP . Smooth means nonsingular.) n n Varieties (at their most basic, algebraic subsets of C or CP . 2 Can have singularities, e.g. -
RING THEORY 1. Ring Theory a Ring Is a Set a with Two Binary Operations
CHAPTER IV RING THEORY 1. Ring Theory A ring is a set A with two binary operations satisfying the rules given below. Usually one binary operation is denoted `+' and called \addition," and the other is denoted by juxtaposition and is called \multiplication." The rules required of these operations are: 1) A is an abelian group under the operation + (identity denoted 0 and inverse of x denoted x); 2) A is a monoid under the operation of multiplication (i.e., multiplication is associative and there− is a two-sided identity usually denoted 1); 3) the distributive laws (x + y)z = xy + xz x(y + z)=xy + xz hold for all x, y,andz A. Sometimes one does∈ not require that a ring have a multiplicative identity. The word ring may also be used for a system satisfying just conditions (1) and (3) (i.e., where the associative law for multiplication may fail and for which there is no multiplicative identity.) Lie rings are examples of non-associative rings without identities. Almost all interesting associative rings do have identities. If 1 = 0, then the ring consists of one element 0; otherwise 1 = 0. In many theorems, it is necessary to specify that rings under consideration are not trivial, i.e. that 1 6= 0, but often that hypothesis will not be stated explicitly. 6 If the multiplicative operation is commutative, we call the ring commutative. Commutative Algebra is the study of commutative rings and related structures. It is closely related to algebraic number theory and algebraic geometry. If A is a ring, an element x A is called a unit if it has a two-sided inverse y, i.e. -
1 Semifields
1 Semifields Definition 1 A semifield P = (P; ⊕; ·): 1. (P; ·) is an abelian (multiplicative) group. 2. ⊕ is an auxiliary addition: commutative, associative, multiplication dis- tributes over ⊕. Exercise 1 Show that semi-field P is torsion-free as a multiplicative group. Why doesn't your argument prove a similar result about fields? Exercise 2 Show that if a semi-field contains a neutral element 0 for additive operation and 0 is multiplicatively absorbing 0a = a0 = 0 then this semi-field consists of one element Exercise 3 Give two examples of non injective homomorphisms of semi-fields Exercise 4 Explain why a concept of kernel is undefined for homorphisms of semi-fields. A semi-field T ropmin as a set coincides with Z. By definition a · b = a + b, T rop a ⊕ b = min(a; b). Similarly we define T ropmax. ∼ Exercise 5 Show that T ropmin = T ropmax Let Z[u1; : : : ; un]≥0 be the set of nonzero polynomials in u1; : : : ; un with non- negative coefficients. A free semi-field P(u1; : : : ; un) is by definition a set of equivalence classes of P expression Q , where P; Q 2 Z[u1; : : : ; un]≥0. P P 0 ∼ Q Q0 if there is P 00;Q00; a; a0 such that P 00 = aP = a0P 0;Q00 = aQ = a0Q0. 1 0 Exercise 6 Show that for any semi-field P and a collection v1; : : : ; vn there is a homomorphism 0 : P(u1; : : : ; un) ! P ; (ui) = vi Let k be a ring. Then k[P] is the group algebra of the multiplicative group of the semi-field P.