In Vitro Bioactivity and Cell Biocompatibility of a Hypereutectic Bioceramic
Total Page:16
File Type:pdf, Size:1020Kb
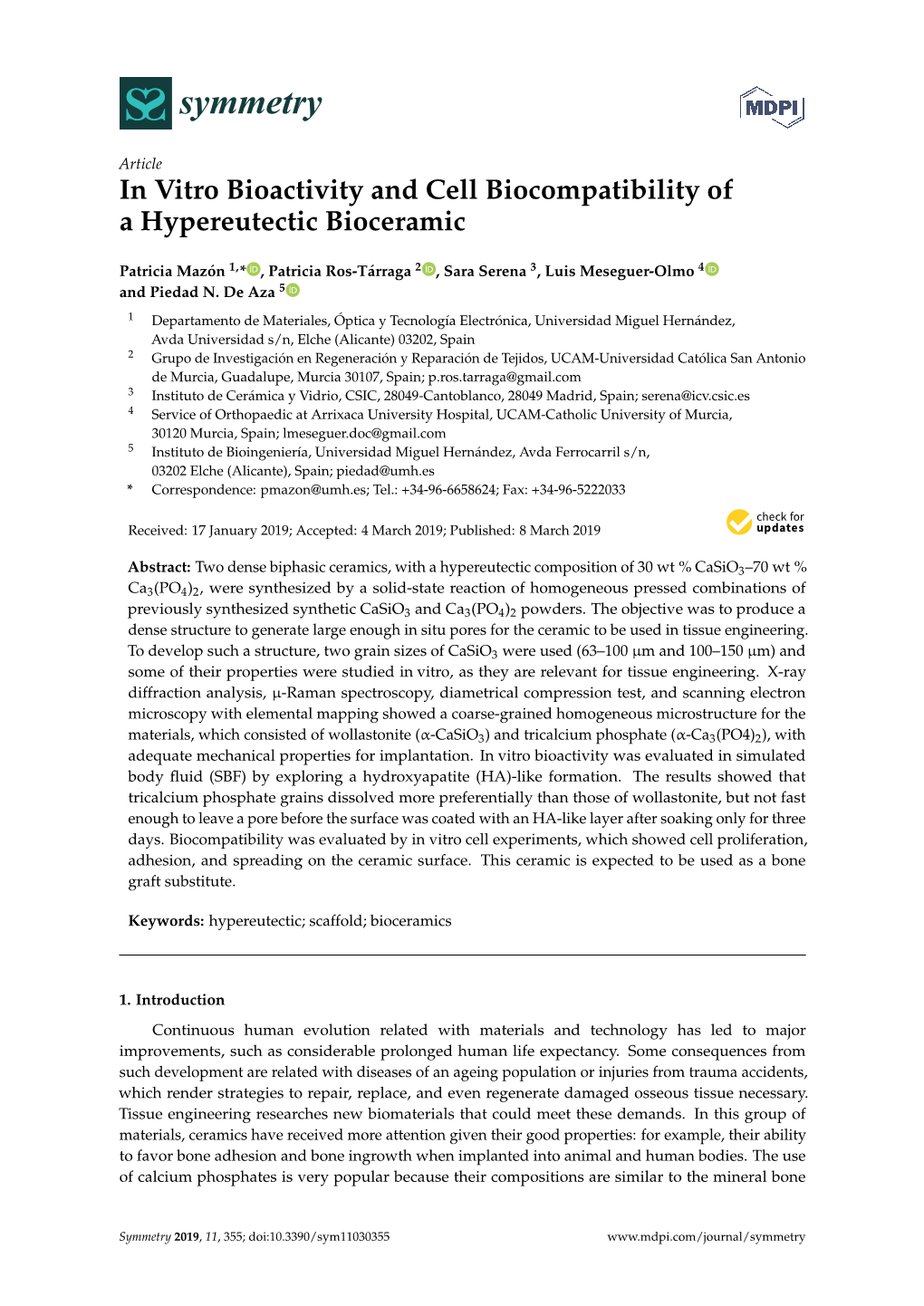
Load more
Recommended publications
-
Metal Oxide Nanostructures and Their Applications (ISBN 1-58883-170-1)
Metal Oxide Nanostructures and their Applications (ISBN 1-58883-170-1) Edited by Ahmad Umar, Najran University, Saudi Arabia Yoon-Bong Hahn, Chonbuk National University, South Korea. Published by American Scientific Publishers, CA, USA, (http://www.aspbs.com/mona/) October 2009 5-Volume Set, 4,000 pages, Hardcover Volume 4 Applications (Part-2) Chapter 13 Bioactive Bioceramic Coatings. Part II: Coatings on Metallic Biomaterials (Vol.4, pp 479-509) Proof-reading Corrections: No. Original Correction 1. Reference [39] 39. A. K. Lynn and D. L. DuQuesnay, Biomaterials 23, 1947 (2002). 2. Reference [97] 97. M. Wei, A. J. Ruys, B. K. Milthorpe, C. C. Sorrell, and J. H. Evans, J. Sol–Gel Sci. Techn. 21, 39 (2001). 3. Figure 6b ⊕ PO ∇ CO Titania 4 3 48 h ⊕ ∇ ⊕ ⊕ ∇∇ ⊕ ⊕ ⊕ ⊕ ⊕ ∇ 24 h ∇ ⊕⊕ Reflectance /arb. units /arb. Reflectance 0 h 2000 1600 1200 800 400 Wavelength /cm-1 CHAPTER 13 Bioactive Bioceramic Coatings: Part II. Coatings on Metallic Biomaterials Jin-Ming Wu1,Min Wang 2 1Department of Materials Science and Engineering, Zhejiang University, Hangzhou 310027, P. R. China 2Department of Mechanical Engineering, The University of Hong Kong, Pokfulam Road, Hong Kong CONTENTS 1. Introduction ..................................... 2 2. Bioactive Bioceramic Coatings ....................... 2 2.1. Physical Deposition ........................... 2 2.2. Sol–Gel Process .............................. 5 2.3. Electrocrystallization, Electrophoretic Deposition, and Electrostatic Spray Deposition ................ 6 2.4. Biomimetic Process ........................... 7 2.5. Other Coating Techniques ..................... 10 3. Nanostructured Titania for In Vitro Apatite Deposition .... 10 3.1. Coating Approaches ......................... 11 3.2. Chemical Modification Approaches .............. 14 4. Composite Coatings .............................. 20 4.1. Ceramic/Metal Composite Coatings ............. 20 4.2. -
Bioactive Glasses: from Parent 45S5 Composition to Scaffold-Assisted Tissue-Healing Therapies
Journal of Functional Biomaterials Review Bioactive Glasses: From Parent 45S5 Composition to Scaffold-Assisted Tissue-Healing Therapies Elisa Fiume, Jacopo Barberi, Enrica Verné * and Francesco Baino * ID Institute of Materials Physics and Engineering, Department of Applied Science and Technology, Politecnico di Torino, Corso Duca degli Abruzzi 24, 10129 Torino, Italy; elisa.fi[email protected] (E.F.); [email protected] (J.B.) * Correspondence: [email protected] (E.V.); [email protected] (F.B.); Tel.: +39-011-090-4717 (E.V.); +39-011-090-4668 (F.B.) Received: 19 February 2018; Accepted: 13 March 2018; Published: 16 March 2018 Abstract: Nowadays, bioactive glasses (BGs) are mainly used to improve and support the healing process of osseous defects deriving from traumatic events, tumor removal, congenital pathologies, implant revisions, or infections. In the past, several approaches have been proposed in the replacement of extensive bone defects, each one with its own advantages and drawbacks. As a result, the need for synthetic bone grafts is still a remarkable clinical challenge since more than 1 million bone-graft surgical operations are annually performed worldwide. Moreover, recent studies show the effectiveness of BGs in the regeneration of soft tissues, too. Often, surgical criteria do not match the engineering ones and, thus, a compromise is required for getting closer to an ideal outcome in terms of good regeneration, mechanical support, and biocompatibility in contact with living tissues. The aim of the present review is providing a general overview of BGs, with particular reference to their use in clinics over the last decades and the latest synthesis/processing methods. -
Injectable Bioactive Glass in the Restoration of Oral Bone Defect
European Review for Medical and Pharmacological Sciences 2016; 20: 1665-1668 Injectable bioactive glass in the restoration of oral bone defect C.-B. HAN, S.-C. AN Department of Oral and Maxillofacial Surgery, Weifang People’s Hospital, Weifang, Shandong, China Abstract. – OBJECTIVE: To explore the appli- the optimal graft material, but these materials had cation value of injectable bioactive glass in the some limitations2. The injectable bioactive glass had restoration of the oral bone defect. good bioactivity and biocompatibility, ion exchange PATIENTS AND METHODS: This study includ- that occurred between the bioactive glass and soft ed 58 consecutive patients with oral bone defect > 1 mm, these patients were randomly assigned tissue as well as bone may be directly involved in to a control group (n=26, Hydroxyapatite bioce- the metabolism and restoration of human bone tis- ramics) and an observation group (n=32, Inject- sue. As a result, identical inorganic mineral, i.e., car- able bioactive glass). The purpose of this study bonated hydroxyapatite could form on the material was to assess the comparison of the healing of surface, inducing growth of new bone tissue3,4. Most oral bone defect. previous studies explored the application value of RESULTS: X-ray examination was performed at 5,6 6-month and 12-month following treatment. The bioactive glass through animal models , while this bone healing in the observation group was sig- study would further confirm the value of bioactive nificantly better than the control group (p <0.05), glass through clinical controlled trial in humans. the incidences of local rejection reactions were not significantly different (p >0.05). -
Current Progress in Solution Precursor Plasma Spraying of Cermets: a Review
metals Review Current Progress in Solution Precursor Plasma Spraying of Cermets: A Review Romnick Unabia 1,2,*, Rolando Candidato Jr. 1 and Lech Pawłowski 2 ID 1 Physics Department, College of Science and Mathematics, MSU-Iligan Institute of Technology, Iligan City 9200, Philippines; [email protected] 2 IRCER UMR 7315 CNRS, University of Limoges, Limoges Cedex 87068, France; [email protected] * Correspondence: [email protected]; Tel.: +33-0-587-502-400 Received: 1 May 2018; Accepted: 1 June 2018; Published: 4 June 2018 Abstract: Ceramic and metal composites, known also as cermets, may considerably improve many material properties with regards to that of initial components. Hence, cermets are frequently applied in many technological fields. Among many processes which can be employed for cermet manufacturing, thermal spraying is one of the most frequently used. Conventional plasma spraying of powders is a popular and cost-effective manufacturing process. One of its most recent innovations, called solution precursor plasma spraying (SPPS), is an emerging coating deposition method which uses homogeneously mixed solution precursors as a feedstock. The technique enables a single-step deposition avoiding the powder preparation procedures. The nanostructured coatings developed by SPPS increasingly find a place in the field of surface engineering. The present review shows the recent progress in the fabrication of cermets using SPPS. The influence of starting solution precursors, such as their chemistry, concentration, and solvents used, to the micro-structural characteristics of cermet coatings is discussed. The effect of the operational plasma spray process parameters such as solution injection mode to the deposition process and coatings’ microstructure is also presented. -
Silicon Nitride, a Close to Ideal Ceramic Material for Medical Application
ceramics Review Silicon Nitride, a Close to Ideal Ceramic Material for Medical Application Robert B. Heimann Am Stadtpark 2A, D-02826 Görlitz, Germany; [email protected]; Tel.: +49-3581-667851 Abstract: This topical review describes the salient results of recent research on silicon nitride, a ceramic material with unique properties. The outcome of this ongoing research strongly encourages the use of monolithic silicon nitride and coatings as contemporary and future biomaterial for a variety of medical applications. Crystallographic structure, the synthesis and processing of monolithic structures and coatings, as well as examples of their medical applications that relate to spinal, orthopedic and dental implants, bone grafts and scaffolds, platforms for intelligent synthetic neural circuits, antibacterial and antiviral particles and coatings, optical biosensors, and nano-photonic waveguides for sophisticated medical diagnostic devices are all covered in the research reviewed herein. The examples provided convincingly show that silicon nitride is destined to become a leader to replace titanium and other entrenched biomaterials in many fields of medicine. Keywords: silicon nitride; structure; properties; processing; coatings; spinal implants; arthroplastic implants; bone scaffolds; dental implants; neural circuits; biosensors; medical diagnostics Citation: Heimann, R.B. Silicon 1. Introduction Nitride, a Close to Ideal Ceramic Material for Medical Application. Today, the research and development of metallic, ceramic, polymeric and composite Ceramics 2021, 4, 208–223. biomaterials have progressed to a high level of involvement and sophistication. This is https://doi.org/10.3390/ related to the fact that an ever-increasing part of the world population is in need of the repair ceramics4020016 or replacement of dysfunctional or damaged parts of the body, such as dental roots and teeth, alveolar ridge augmentation, intraocular lenses, heart pacemakers, cochlear implants, Academic Editors: Stuart Hampshire and hip and knee endoprostheses [1]. -
Bioceramic in Dental Implants: a Review
Journal of Indian Prosthodontic Society (March 2010) 10:8–12 8DOI: 10.1007/s13191-010-0002-4 Journal of Indian Prosthodontic Society (March 2010) 10:8–12 REVIEW ARTICLE Bioceramic in Dental Implants: A Review Gaurav P. Jayaswal, S. P. Dange, A. N. Khalikar © Indian Prosthodontic Society Biomaterials are non-drug substance suitable for inclusion in system which augment or replace the function of bodily tissue or organ. Orthopedic and dental applications represent approximately 55% of the total biomaterials market. Changes in biologic responses and device design have been the direct result of advances in material science. Bioceramics fulfi ll a unique function as biomedical materials. Bioceramics are non-toxic and bioinert, bioactive or bioresorbable. Bioceramics continue to be vital for bone repair and uncemented implant fi xation with recent advances in its composition and coating technology. Keywords: Biomaterials, Hydroxyapatite, Zirconia Introduction responses and device design have been the direct result of advances in material science. An implant is defi ned as a medical device made from one Bioceramics primarily have provided the research and or more biomaterials that is intentionally placed within the dental clinical professionals with new substances of interest body, either totally or partially buried beneath an epithelial for over last 2 decades. Various formulations of these surface. Biomaterials by defi nation is a non-drug substance materials have been conceived on the basis of their chemical suitable for inclusion in system which augment or replace the similarity to bone. function of bodily tissue or organ. From as early as a century ago artifi cial material and device have been developed to a point where they can replace various component of the Bioceramics human body. -
Preparation and Evaluation of Bioceramic Composite Based on Hydroxyapatite and Titania Nanoparticles for Biomedical Applications Ibrahim
International Journal of Scientific & Engineering Research, Volume 7, Issue 4, April-2016 641 ISSN 2229-5518 Preparation and evaluation of Bioceramic composite based on hydroxyapatite and titania nanoparticles for biomedical applications Ibrahim. H. M. Alya, Nasser A. M. Barakata,b, L. Abed Alrahim Mohammedc Abstract—in this study, composite material comprised of titanium dioxide (TiO2) nanoparticles incorporated with bone derived hydroxyapatite was prepared to investigate the possibility of using it as bone substitute in high load bearing sites. The structure and phase composition of the produced sintered bodies were characterized by X-ray diffraction (XRD) and scanning electron microscopy (SEM). Densification rate was evaluated by measuring apparent porosity. A mechanical characterization of the sintered samples was carried out by means of Vickers indentations and compressive strength determinations. The bioactivity was assessed by investigating the formation of apatite on the film surface after soaking in simulated body fluid. The results showed that the proposed composite present higher densification, better mechanical characteristics compared to pure hydroxyapatite. Moreover, the results also showed bone like apatite was formed on the surface of hydroxyapatite/TiO2 nanoparticles soaked in simulated body fluid. The results obtained demonstrate that hydroxyapatite can be considered suitable as filler in biomedical applications while glass-ceramic matrix composite reinforced with titania nanostructure can be used in high load bearing applications from the point of view of the biomechnical properties. This behavior suggests a possibility of a further favorable in vivo response. Index Terms— Bioceramics, biomaterial, Bovine hydroxyapatite, Mechanical properties, In vitro study, simulated body fluid (SBF), titania nanoparticles ————————— —————————— 1. Introduction metals, polymers, calcium phosphate biomaterials, bioglasses have been studied for bone repair [1-6]. -
Bioceramics for Hard Tissue Engineering Applications: a Review
International Journal of Applied Engineering Research ISSN 0973-4562 Volume 13, Number 5 (2018) pp. 2744-2752 © Research India Publications. http://www.ripublication.com Bioceramics for Hard Tissue Engineering Applications: A Review Pawan Kumar1,*, Brijnandan S Dehiya1 and Anil Sindhu2 1Department of Materials Science and Nanotechnology 2Department of Biotechnology Deenbandhu Chhotu Ram University of Science and Technology, Sonipat-131039, India. Email: [email protected] Abstract tissue engineering [3]. In the early years, the focus on anti- infective or antibacterial biomaterials synthesis has been The bioceramics are using as a substitute for hard tissue increasing. It helps to prevent scaffolds or implants from engineering since 1960. A compelling way to deal with hard undesirable infection and keep sterile. It also reduces the risk tissue engineering aims to restore function of diseased or rate of infection during special surgery cases [4]. In cases of damaged bone tissue by combining isolated functional cells and open fractured joints and bone modification surgeries, the biodegradable scaffolds prepared from engineered implants can lead to the microbial disease [5]. The microbe’s bioceramics. Bioceramic materials having better potential to species attached on the surface of materials through different replace the damaged tissues and restore the function of existing mechanisms. According to early stated mechanism, microbe’s tissue. ZrO , Al O based materials and composites presently 2 2 3 adhesion done by passive adsorption through which bacterial used to fabricate implants. Bioceramics including cells exposed to a solid surface of biomaterials, which offers hydroxyapatite, bioglass, and calcium phosphate are using as surface interactions between materials and bacterial cells [6]. -
Additive Manufacturing of Hydroxyapatite Bioceramic Scaffolds: Dispersion, Digital Light Processing, Sintering, Mechanical Properties, and Biocompatibility
Journal of Advanced Ceramics 2020, 9(3): 360–373 ISSN 2226-4108 https://doi.org/10.1007/s40145-020-0375-8 CN 10-1154/TQ Research Article Additive manufacturing of hydroxyapatite bioceramic scaffolds: Dispersion, digital light processing, sintering, mechanical properties, and biocompatibility Chengwei FENGa, Keqiang ZHANGa, Rujie HEa,*, Guojiao DINGa, Min XIAb, Xinxin JINc, Chen XIEd,* aInstitute of Advanced Structure Technology, Beijing Institute of Technology, Beijing 100081, China bSchool of Materials Science and Engineering, Beijing Institute of Technology, Beijing 100081, China cSchool of Materials Science and Engineering, Harbin University of Science and Technology, Harbin 150040, China dShanghai Aircraft Manufacturing Co., Ltd., Shanghai 201324, China Received: October 21, 2019; Revised: February 3, 2020; Accepted: March 18, 2020 © The Author(s) 2020. Abstract: Hydroxyapatite (HA) bioceramic scaffolds were fabricated by using digital light processing (DLP) based additive manufacturing. Key issues on the HA bioceramic scaffolds, including dispersion, DLP fabrication, sintering, mechanical properties, and biocompatibility were discussed in detail. Firstly, the effects of dispersant dosage, solid loading, and sintering temperature were studied. The optimal dispersant dosage, solid loading, and sintering temperature were 2 wt%, 50 vol%, and 1250 ℃, respectively. Then, the mechanical properties and biocompatibility of the HA bioceramic scaffolds were investigated. The DLP-prepared porous HA bioceramic scaffold was found to exhibit excellent mechanical properties and degradation behavior. From this study, DLP technique shows good potential for manufacturing HA bioceramic scaffolds. Keywords: additive manufacturing; digital light processing; vat photopolymerization; hydroxyapatite; bioceramic scaffold and bioactivity [2–4]. The excellent osteoconductivity 1 Introduction makes HA as an artificial substitute material for bone, and also makes bone cells adhere, proliferate, and Bioceramics have attracted great attentions in recent mineralize on surface. -
Improvement of Reparative Bioceramics in Endodontics - a Critical Review
Mini Review ISSN: 2574 -1241 DOI: 10.26717/BJSTR.2020.24.004059 Improvement of Reparative Bioceramics in Endodontics - A Critical Review Stella Maris de Freitas Lima1*, Poliana Amanda Oliveira Silva2 and Taia Maria Berto Rezende1,2,3 1Dental Course, Universidade Católica de Brasília, Brazil 2 Post graduation in Health Sciences, Universidade de Brasília, Brazil 3Post graduation in Genomic Sciences and Biotechnology, Universidade Católica de Brasília, Brazil *Corresponding author: Stella Maris de Freitas Lima, Professor of Endodontics, Universidade Católica de Brasília, Brazil ARTICLE INFO Abstract Received: December 30, 2019 The advent of bioceramics materials increases the possibility of reparative and Published: January 10, 2020 regenerative processes in dentistry, and more precisely, in endodontics. Bioceramics materials presents biocompatibility, bioactivity, resistance to leakage, sealing ability, and biomineralization activity. Mineral trioxide aggregate (MTA) is a gold standard Citation: Stella Maris de Freitas Lima, bioceramic material indicated for reparative procedures presenting high clinical Poliana Amanda Oliveira Silva, Taia Maria Berto Rezende. Improvement of Repara- resorption repair, regenerative endodontics, and apical surgery. The emergence of success. MTA is used for pulp capping, pulpotomy, apexification, perforation, root tive Bioceramics in Endodontics - A Critical improvements of this bioceramic gave rise to many other materials. New bioceramic Review. Biomed J Sci & Tech Res 24(3)- materialsMTA demonstrated are -
Characteristics, Preparation and Improvement of Porous Hydroxyapatite Bioceramic Materials
id247101593 pdfMachine by Broadgun Software - a great PDF writer! - a great PDF creator! - http://www.pdfmachine.com http://www.broadgun.com BBiiooTTISSNe e: 097cc4 - h7h435 nnooVlolluoome 8g gIssueyy 11 An Indian Journal FULL PAPER BTAIJ, 8(11), 2013 [1463-1470] Characteristics, preparation and improvement of porous hydroxyapatite bioceramic materials Yanrong Sun, Tao Fan*, Liguo Ma, Qiuxia, Zhang, Hongbo Li, Feng Liu Department of Materials Engineering, North China Institute of Aerospace Engineering, Langfang065000, (CHINA) E-mail: [email protected] ABSTRACT KEYWORDS Hydroxyapatite exhibits the outstanding biocompatibility and bioactivity Porous; with bone replacement in living tissues. Porous hydroxyapatite bioceramic Hydroxyapatite; is able to intensity the osteoconduction and osteoinduction and suitable Bioceramic. for artificial bone substitutes. By means of controllingporosity, size, interconnectivity and surface roughness of pores, the porous HA with optimal properties can be obtained. Variousmethods for preparing porous HA bioceramic have been introduced, such as pore-forming agent method, foaming method, conversion of natural body, colloidal template method, and freeze casting. To improve properties of porous HA bioceramic, two or several kinds of preparation methodshave beencombined to use, and designing and developing porous HA-based biocomposite materials is also acceptable. 2013 Trade Science Inc. - INDIA INTRODUCTION focus on preparing porous HA ceramics, tailoring the morphology of HA powders, and Designing HA com- [6] Hydroxyapatite ([Ca10(PO4)6(OH)2], HA or HAP), posites . Porous HA have been applied for cell load- one of the most representational bioceramic, which ex- ing, drug releasing agents, chromatography analysis, and hibits the outstanding biocompatibility and bioactivity the most extensively for hard tissue scaffolds[7,8]. -
Coehlo P, Bromage TG. Challenges In
Chapter 1 The Challenges in Engineering and Testing Dental Bioceramics by Paulo Coelho and Timothy G. Bromage Introduction The clinical success of modern dental ceramics is predicated on a number of factors, such as the initial physical properties of these brittle materials, the fabrication and clinical procedures that unavoidably damage them, and the oral environment. Examining how these factors influence clinical performance has involved investigators from the dental, ceramics, and engineering communities. This chapter will first summarize the rationale behind engineering biomimetic ceramic dental restorative materials, then critically describe the basic methodology and analytic results reported in recent literature. Basic Properties of Enamel and Dentin as Objectives for Bioceramic Development There are several important properties of tooth structure relevant to bioceramic biomimetic design. Paramount is (1) mechanical efficacy, largely a function of tooth crown morphology, enamel thickness and microstructure, and root structure. Related to this is (2) proprioception, the internal perceptions that allow us to reduce the probability of catastrophic fracture from excessive loads transmitted through enamel into dentine and the supporting structures. An additional consideration is (3) the porosity of healthy enamel. Further, it is fair to say that people who take their oral care seriously enough to be receiving bioceramic implants will also be concerned with (4) the aesthetics of their natural teeth. Finally, if properly managed, enamel