S7. 'Zptt Date
Total Page:16
File Type:pdf, Size:1020Kb
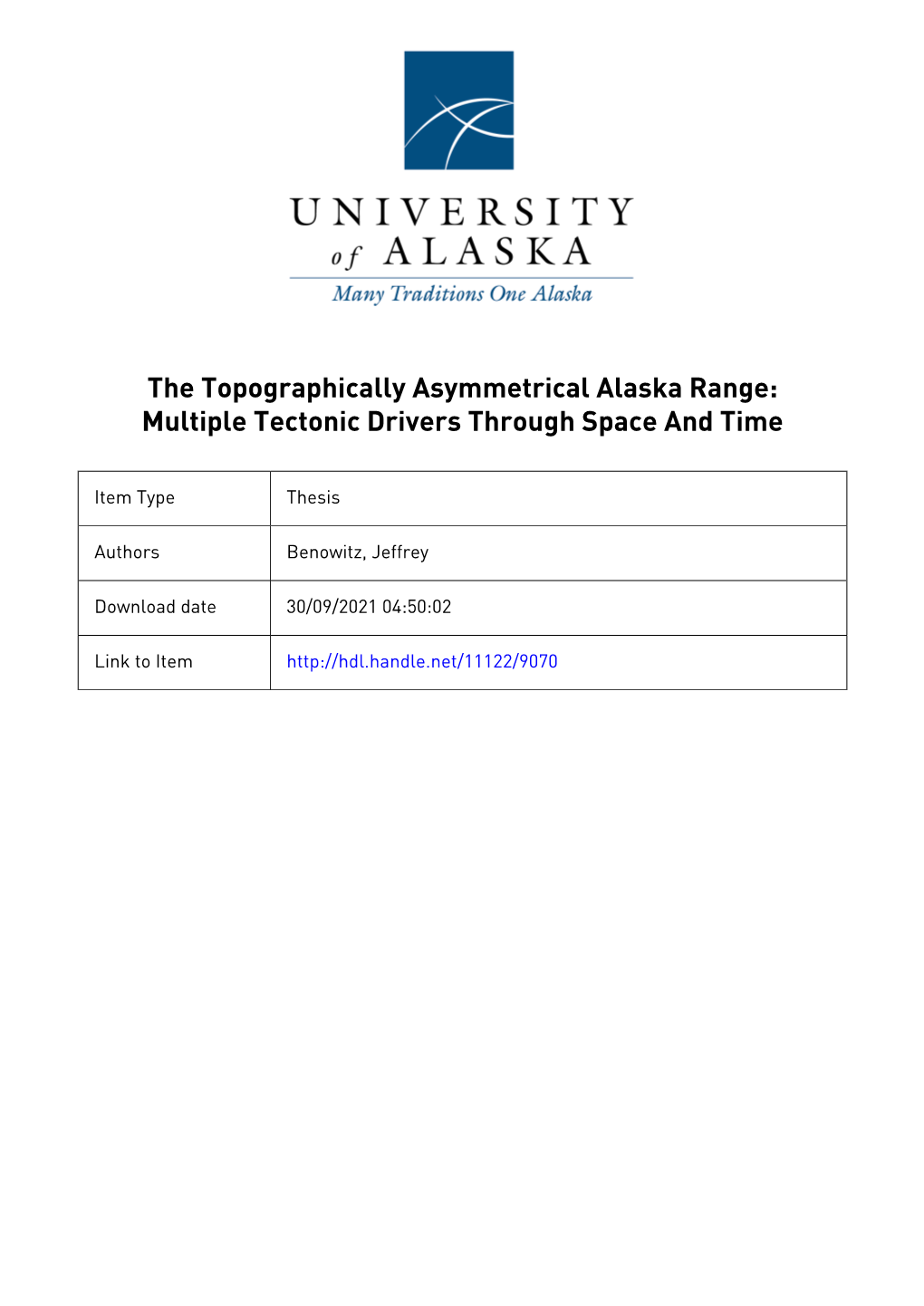
Load more
Recommended publications
-
Alaska Division of Geological & Geophysical Surveys Geologic
Cretaceous to Tertiary magmatism and associated mineralization in the Lime Hills C-1 Quadrangle, Western Alaska Range Karri R. Sicard 1, Evan Twelker1, Larry K. Freeman1, Alicja Wypych1, Jeff A. Benowitz2 , and M. Andy Kass3 1Alaska Division of Geological & Geophysical Surveys, Fairbanks, Alaska 2Geophysical Institute, Department of Geology & Geophysics, University of Alaska Fairbanks 3Crustal Geophysics and Geochemistry Science Center, US Geological Survey, Denver, CO Alaska Miners Association Annual Convention 1 November 5, 2014 – Anchorage, Alaska Outline ► Location and geology ► Mineralization highlights ► New 40Ar/39Ar geochronology . With relevant geochemistry ► 3D voxel model of Copper Joe resistivity ► Relationship to Revelation Mountains uplift ► Acknowledgments 2 Photo by T.C. Wright Cretaceous-Tertiary Porphyry Trend ~70 Ma Select Northern Cordillera ~90 Ma porphyry copper deposits ~6 Ma 3 http://www.kiskametals.com Terrane Map YUKON-TANANA TERRANE McGrath WRANGELLIA Project Area Anchorage 4 Modified from Colpron and Nelson, 2011 Denali-Farewell Fault System Jurassic-Cretaceous Revelation flysch Mountains Lime Hills C-1 Cretaceous and Tertiary Quad plutonic rocks Tordrillo Mountains Tertiary volcanics 5 Map modified from Wilson and others, 1998 Airborne Geophysical Surveys Farewell (Burns and others, 2014) Middle Styx (Burns and others, 2013) East Styx More positive magnetic values (Released November 2014) Styx Lime Hills (Burns and More negative others 2008) 6 magnetic values C-1 Quad nT Anomalous Gold Occurrences Circles -
Geologic Maps of the Eastern Alaska Range, Alaska, (44 Quadrangles, 1:63360 Scale)
Report of Investigations 2015-6 GEOLOGIC MAPS OF THE EASTERN ALASKA RANGE, ALASKA, (44 quadrangles, 1:63,360 scale) descriptions and interpretations of map units by Warren J. Nokleberg, John N. Aleinikoff, Gerard C. Bond, Oscar J. Ferrians, Jr., Paige L. Herzon, Ian M. Lange, Ronny T. Miyaoka, Donald H. Richter, Carl E. Schwab, Steven R. Silva, Thomas E. Smith, and Richard E. Zehner Southeastern Tanana Basin Southern Yukon–Tanana Upland and Terrane Delta River Granite Jarvis Mountain Aurora Peak Creek Terrane Hines Creek Fault Black Rapids Glacier Jarvis Creek Glacier Subterrane - Southern Yukon–Tanana Terrane Windy Terrane Denali Denali Fault Fault East Susitna Canwell Batholith Glacier Maclaren Glacier McCallum Creek- Metamorhic Belt Meteor Peak Slate Creek Thrust Broxson Gulch Fault Thrust Rainbow Mountain Slana River Subterrane, Wrangellia Terrane Phelan Delta Creek River Highway Slana River Subterrane, Wrangellia Terrane Published by STATE OF ALASKA DEPARTMENT OF NATURAL RESOURCES DIVISION OF GEOLOGICAL & GEOPHYSICAL SURVEYS 2015 GEOLOGIC MAPS OF THE EASTERN ALASKA RANGE, ALASKA, (44 quadrangles, 1:63,360 scale) descriptions and interpretations of map units Warren J. Nokleberg, John N. Aleinikoff, Gerard C. Bond, Oscar J. Ferrians, Jr., Paige L. Herzon, Ian M. Lange, Ronny T. Miyaoka, Donald H. Richter, Carl E. Schwab, Steven R. Silva, Thomas E. Smith, and Richard E. Zehner COVER: View toward the north across the eastern Alaska Range and into the southern Yukon–Tanana Upland highlighting geologic, structural, and geomorphic features. View is across the central Mount Hayes Quadrangle and is centered on the Delta River, Richardson Highway, and Trans-Alaska Pipeline System (TAPS). Major geologic features, from south to north, are: (1) the Slana River Subterrane, Wrangellia Terrane; (2) the Maclaren Terrane containing the Maclaren Glacier Metamorphic Belt to the south and the East Susitna Batholith to the north; (3) the Windy Terrane; (4) the Aurora Peak Terrane; and (5) the Jarvis Creek Glacier Subterrane of the Yukon–Tanana Terrane. -
Club Activities
Club Activities EDITEDBY FREDERICKO.JOHNSON A.A.C.. Cascade Section. The Cascade Section had an active year in 1979. Our Activities Committee organized a slide show by the well- known British climber Chris Bonington with over 700 people attending. A scheduled slide and movie presentation by Austrian Peter Habeler unfortunately was cancelled at the last minute owing to his illness. On-going activities during the spring included a continuation of the plan to replace old bolt belay and rappel anchors at Peshastin Pinnacles with new heavy-duty bolts. Peshastin Pinnacles is one of Washington’s best high-angle rock climbing areas and is used heavily in the spring and fall by climbers from the northwestern United States and Canada. Other spring activities included a pot-luck dinner and slides of the American Women’s Himalayan Expedition to Annapurna I by Joan Firey. In November Steve Swenson presented slides of his ascents of the Aiguille du Triolet, Les Droites, and the Grandes Jorasses in the Alps. At the annual banquet on December 7 special recognition was given to sec- tion members Jim Henriot, Lynn Buchanan, Ruth Mendenhall, Howard Stansbury, and Sean Rice for their contributions of time and energy to Club endeavors. The new chairman, John Mendenhall, was introduced, and a program of slides of the alpine-style ascent of Nuptse in the Nepal Himalaya was presented by Georges Bettembourg, followed by the film, Free Climb. Over 90 members and guests were in attendance. The Cascade Section Endowment Fund Committee succeeded in raising more than $5000 during 1979, to bring total donations to more than $12,000 with 42% of the members participating. -
2020 January Scree
the SCREE Mountaineering Club of Alaska January 2020 Volume 63, Number 1 Contents Mount Anno Domini Peak 2330 and Far Out Peak Devils Paw North Taku Tower Randoism via Rosie’s Roost "The greatest danger for Berlin Wall most of us is not that our aim is too high and we Katmai and the Valley of Ten Thousand Smokes miss it, but that it is too Peak of the Month: Old Snowy low and we reach it." – Michelangelo JANUARY MEETING: Wednesday, January 8, at 6:30 p.m. Luc Mehl will give the presentation. The Mountaineering Club of Alaska www.mtnclubak.org "To maintain, promote, and perpetuate the association of persons who are interested in promoting, sponsoring, im- proving, stimulating, and contributing to the exercise of skill and safety in the Art and Science of Mountaineering." This issue brought to you by: Editor—Steve Gruhn assisted by Dawn Munroe Hut Needs and Notes Cover Photo If you are headed to one of the MCA huts, please consult the Hut Gabe Hayden high on Devils Paw. Inventory and Needs on the website (http://www.mtnclubak.org/ Photo by Brette Harrington index.cfm/Huts/Hut-Inventory-and-Needs) or Greg Bragiel, MCA Huts Committee Chairman, at either [email protected] or (907) 350-5146 to see what needs to be taken to the huts or repaired. All JANUARY MEETING huts have tools and materials so that anyone can make basic re- Wednesday, January 8, at 6:30 p.m. at the BP Energy Center at pairs. Hutmeisters are needed for each hut: If you have a favorite 1014 Energy Court in Anchorage. -
Alaska Range
Alaska Range Introduction The heavily glacierized Alaska Range consists of a number of adjacent and discrete mountain ranges that extend in an arc more than 750 km long (figs. 1, 381). From east to west, named ranges include the Nutzotin, Mentas- ta, Amphitheater, Clearwater, Tokosha, Kichatna, Teocalli, Tordrillo, Terra Cotta, and Revelation Mountains. This arcuate mountain massif spans the area from the White River, just east of the Canadian Border, to Merrill Pass on the western side of Cook Inlet southwest of Anchorage. Many of the indi- Figure 381.—Index map of vidual ranges support glaciers. The total glacier area of the Alaska Range is the Alaska Range showing 2 approximately 13,900 km (Post and Meier, 1980, p. 45). Its several thousand the glacierized areas. Index glaciers range in size from tiny unnamed cirque glaciers with areas of less map modified from Field than 1 km2 to very large valley glaciers with lengths up to 76 km (Denton (1975a). Figure 382.—Enlargement of NOAA Advanced Very High Resolution Radiometer (AVHRR) image mosaic of the Alaska Range in summer 1995. National Oceanic and Atmospheric Administration image mosaic from Mike Fleming, Alaska Science Center, U.S. Geological Survey, Anchorage, Alaska. The numbers 1–5 indicate the seg- ments of the Alaska Range discussed in the text. K406 SATELLITE IMAGE ATLAS OF GLACIERS OF THE WORLD and Field, 1975a, p. 575) and areas of greater than 500 km2. Alaska Range glaciers extend in elevation from above 6,000 m, near the summit of Mount McKinley, to slightly more than 100 m above sea level at Capps and Triumvi- rate Glaciers in the southwestern part of the range. -
North American Notes
268 NORTH AMERICAN NOTES NORTH AMERICAN NOTES BY KENNETH A. HENDERSON HE year I 967 marked the Centennial celebration of the purchase of Alaska from Russia by the United States and the Centenary of the Articles of Confederation which formed the Canadian provinces into the Dominion of Canada. Thus both Alaska and Canada were in a mood to celebrate, and a part of this celebration was expressed · in an extremely active climbing season both in Alaska and the Yukon, where some of the highest mountains on the continent are located. While much of the officially sponsored mountaineering activity was concentrated in the border mountains between Alaska and the Yukon, there was intense activity all over Alaska as well. More information is now available on the first winter ascent of Mount McKinley mentioned in A.J. 72. 329. The team of eight was inter national in scope, a Frenchman, Swiss, German, Japanese, and New Zealander, the rest Americans. The successful group of three reached the summit on February 28 in typical Alaskan weather, -62° F. and winds of 35-40 knots. On their return they were stormbound at Denali Pass camp, I7,3oo ft. for seven days. For the forty days they were on the mountain temperatures averaged -35° to -40° F. (A.A.J. I6. 2I.) One of the most important attacks on McKinley in the summer of I967 was probably the three-pronged assault on the South face by the three parties under the general direction of Boyd Everett (A.A.J. I6. IO). The fourteen men flew in to the South east fork of the Kahiltna glacier on June 22 and split into three groups for the climbs. -
A New Satellite-Derived Glacier Inventory for Western Alaska
Zurich Open Repository and Archive University of Zurich Main Library Strickhofstrasse 39 CH-8057 Zurich www.zora.uzh.ch Year: 2011 A new satellite-derived glacier inventory for western Alaska Le Bris, R ; Paul, F ; Frey, H ; Bolch, T Abstract: Glacier inventories provide the baseline data to perform climate-change impact assessment on a regional scale in a consistent and spatially representative manner. In particular, a more accurate calculation of the current and future contribution to global sea-level rise from heavily glacierized re- gions such as Alaska is much needed. We present a new glacier inventory for a large part of western Alaska (including Kenai Peninsula and the Tordrillo, Chigmit and Chugach mountains), derived from nine Landsat Thematic Mapper scenes acquired between 2005 and 2009 using well-established automated glacier-mapping techniques (band ratio). Because many glaciers are covered by optically thick debris or volcanic ash and partly calve into water, outlines were manually edited in these wrongly classified regions during post-processing. In total we mapped 8830 glaciers (>0.02 km2) with a total area of 16 250 km2. Large parts of the area (47%) are covered by a few (31) large (>100 km2) glaciers, while glaciers less than 1 km2 constitute only 7.5% of the total area but 86% of the total number. We found a strong dependence of mean glacier elevation on distance from the ocean and only a weak one on aspect. Glacier area changes were calculated for a subset of 347 selected glaciers by comparison with the Digital Line Graph outlines from the US Geological Survey. -
Glenn Highway Tok Cutoff (GJ-125 to GJ-0) to Milepost a 160
Map GLENN HIGHWAY • TOK CUTOFF Glenn Highway To Chicken and Eagle © The MILEPOST To Delta Junction (see TAYLOR HIGHWAY section) Key to mileage boxes ver (see ALASKA HIGHWAY section) Tanana Ri miles/kilometres G miles/kilometres Tanacross 5 from: la A L c A Swb T-Tok V-Valdez ia S K 2 Tok Map Location G-Glennallen ted A ® GJ-Gakona Junction A re A-Anchorage a R 2 A 1 Tetlin Junction J-Junction N HJ-Haines Junction Mount Kimball G T-0 a To Haines DJ-Delta Junction in 10,300 ft./3,139m E ch r w GJ-125/201km Junction Chisto cie G la A-328/528km (see ALASKA Principal Route Logged Key to Advertiser er HIGHWAY Services T iv DJ-108/174km C -Camping ok R section) Paved Unpaved R HJ-296/476km D -Dump Station iv ok Other Roads Logged d -Diesel er T G -Gas (reg., unld.) Tetlin I -Ice Lake Other Roads Scenic Byway L -Lodging M -Meals T Refer to Log for Visitor Facilities P -Propane Tok Cutoff ok Cu L R -Car Repair (major) na i Scale Sla R Mineral Lakes t iv t r -Car Repair (minor) e l 0 10 Miles r e S -Store (grocery) 0 10 Kilometres T -Telephone (pay) . t Cr t Bartell off (GJ-125 r Mentasta Lake e r e t T r. o v iv C i R Mentasta Lake S t ation k R Mentasta Summit n T-65/105km 2,434 ft./742m M a . i r E N d J-0 t C T A n e S I . -
Catalogue 48: June 2013
Top of the World Books Catalogue 48: June 2013 Mountaineering Fiction. The story of the struggles of a Swiss guide in the French Alps. Neate X134. Pete Schoening Collection – Part 1 Habeler, Peter. The Lonely Victory: Mount Everest ‘78. 1979 Simon & We are most pleased to offer a number of items from the collection of American Schuster, NY, 1st, 8vo, pp.224, 23 color & 50 bw photos, map, white/blue mountaineer Pete Schoening (1927-2004). Pete is best remembered in boards; bookplate Ex Libris Pete Schoening & his name in pencil, dj w/ edge mountaineering circles for performing ‘The Belay’ during the dramatic descent wear, vg-, cloth vg+. #9709, $25.- of K2 by the Third American Karakoram Expedition in 1953. Pete’s heroics The first oxygenless ascent of Everest in 1978 with Messner. This is the US saved six men. However, Pete had many other mountain adventures, before and edition of ‘Everest: Impossible Victory’. Neate H01, SB H01, Yak H06. after K2, including: numerous climbs with Fred Beckey (1948-49), Mount Herrligkoffer, Karl. Nanga Parbat: The Killer Mountain. 1954 Knopf, NY, Saugstad (1st ascent, 1951), Mount Augusta (1st ascent) and King Peak (2nd & 1st, 8vo, pp.xx, 263, viii, 56 bw photos, 6 maps, appendices, blue cloth; book- 3rd ascents, 1952), Gasherburm I/Hidden Peak (1st ascent, 1958), McKinley plate Ex Libris Pete Schoening, dj spine faded, edge wear, vg, cloth bookplate, (1960), Mount Vinson (1st ascent, 1966), Pamirs (1974), Aconcagua (1995), vg. #9744, $35.- Kilimanjaro (1995), Everest (1996), not to mention countless climbs in the Summarizes the early attempts on Nanga Parbat from Mummery in 1895 and Pacific Northwest. -
City of Wasilla Hazard Mitigation Plan (Phase I – Natural Hazards)
City of Wasilla Hazard Mitigation Plan (Phase I – Natural Hazards) 2018 Update by: Wasilla Planning Commission Acknowledgements Wasilla City Council Bert Cottle, Mayor Glenda Ledford Tim Burney Stu Graham Mike Dryden Gretchen O’Barr James E. Harvey Wasilla Planning Commission Eric Bushnell Darrell L. Breese Jessica Dean Simon Brown Brian L. Mayer City Staff Tina Crawford, City Planner City of Wasilla Planning Office 290 E. Herning Avenue Wasilla, AK 99654 Phone: (907) 373-9020 Fax: (907) 373-9021 E-Mail: [email protected] Consultants LeMay Engineering & Consulting, Inc. Jennifer LeMay, PE, PMP John Farr, EIT 4272 Chelsea Way Anchorage, Alaska 99504 Phone: (907) 350-6061 Email: [email protected]; [email protected] Technical Assistance Brent Nichols, CFM, SHMO, Alaska State DHS&EM The preparation of this plan was financed by funds from a grant from the Alaska Division of Homeland Security and Emergency Management and the Federal Emergency Management Agency. ii City of Wasilla 2018 Hazard Mitigation Plan Acronyms °F Degrees Fahrenheit ADEC Alaska Department of Environmental Conservation AEIC Alaska Earthquake Information Center AFS Alaska Fire Service AHS Alaska Hydrologic Survey APA Approved Pending Adoption AS Alaska State Statute AKST Alaska Standard Time BCA Benefit-Cost Analysis BCR Benefit-Cost Ratio BLM Bureau of Land Management CFR Code of Federal Regulations DCCED Department of Commerce, Community, and Economic Development (State of Alaska) DHS&EM Department of Homeland Security and Emergency Management -
Wejchert 2013 Winner Final
Waterman Fund Essay Contest Winner Epigoni, Revisited The trappings of the digital age follow a climber far north Michael Wejchert Editor’s note: The winner of the sixth annual Waterman Fund essay contest, which Appalachia sponsors jointly with the Waterman Fund, offers an honest look at a climber’s ambivalence toward the technology he uses before and during an attempt to climb Mount Deborah in Alaska. We hope that Michael Wejchert’s approach to the cell phones and Web-based forums we now take for granted will open a new dialogue about the relationship between technology and wilderness. The Waterman Fund is a nonprofit organization named in honor of Laura and the late Guy Waterman. It is our mission to encourage new writers. See the end pages of this journal for information about next year’s contest. The machine does not isolate man from the great problems of nature but plunges him more deeply into them. —Antoine de Saint-Exupéry We are not in New England. Certainly. Caribou tracks end in small dots beneath Paul Roderick’s single-engine DHC-2 Beaver. Denali, where Paul knows glacial landings like city slickers know commuter shortcuts, is more than a hundred miles away. Every once in a while, Paul lets go of the rudder to take a picture, and the plane bucks wildly. Opening the window, offering his camera to the polar air, he snaps photos of mountains without names. Bayard Russell, Elliot Gaddy, and I have all flown with Paul before, but we’ve never seen him like this. We exchange nervous glances. -
DOCUMENT RESUME ED 262 131 UD 024 468 TITLE Hawaiian
DOCUMENT RESUME ED 262 131 UD 024 468 TITLE Hawaiian Studies Curriculum Guide. Grade 3. INSTITUTION Hawaii State Dept. of Education, Honolulu. Office of Instructional Services. PUB DATE Jan 85 NOTE 517p.; For the Curriculum Guides for Grades K-1, 2, and 4, see UD 024 466-467, and ED 255 597. PUB TYPE Guides - Classroom Use - Guides (For Teachers) (052) EDRS PRICE MF02/PC21 Plus Postage. DESCRIPTORS *Cultural Awareness; *Cultural Education; Elementary Education; *Environmental Education; Geography; *Grade 3; *Hawaiian; Hawaiians; Instructional Materials; *Learning Activities; Pacific Americans IDENTIFIERS *Hawaii ABSTRACT This curriculum guide suggests activities and educational experiences within a Hawaiian cultural context for Grade 3 students in Hawaiian schools. First, an introduction discussesthe contents of the guide; the relationship of classroom teacher and the kupuna (Hawaiian-speaking elder); the identification and scheduling of Kupunas; and how to use the guide. The remainder of thetext is divided into two major units. Each is preceded byan overview which outlines the subject areas into which Hawaiian Studies instructionis integrated; the emphases or major lesson topics takenup within each subject area; the learning objectives addressed by the instructional activities; and a key to the unit's appendices, which provide cultural information to supplement the activities. Unit I focuseson the location of Hawaii as one of the many groups of islands in the Pacific Ocean. The learning activities suggestedare intended to teach children about place names, flora and fauna,songs, and historical facts about their community, so that they learnto formulate generalizations about location, adaptation, utilization, and conservation of their Hawaiian environment. Unit II presents activities which immerse children in the study of diverse urban and rural communities in Hawaii.