Asteroid Redirect Mission (ARM) Using Solar Electric Propulsion (SEP) for Research, Mining, and Exploration Endeavors of Near- Earth Objects (Neos)
Total Page:16
File Type:pdf, Size:1020Kb
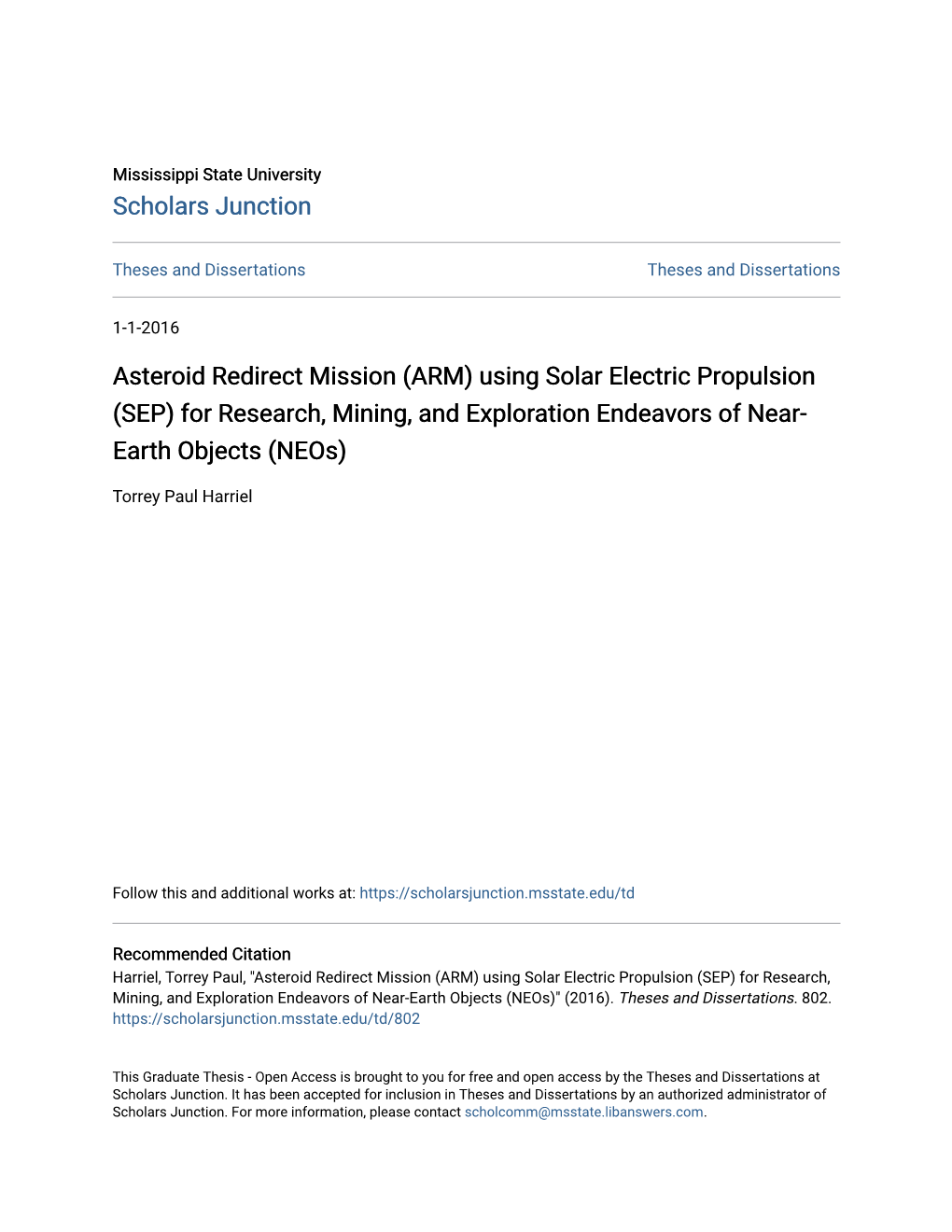
Load more
Recommended publications
-
Russia's Posture in Space
Russia’s Posture in Space: Prospects for Europe Executive Summary Prepared by the European Space Policy Institute Marco ALIBERTI Ksenia LISITSYNA May 2018 Table of Contents Background and Research Objectives ........................................................................................ 1 Domestic Developments in Russia’s Space Programme ............................................................ 2 Russia’s International Space Posture ......................................................................................... 4 Prospects for Europe .................................................................................................................. 5 Background and Research Objectives For the 50th anniversary of the launch of Sputnik-1, in 2007, the rebirth of Russian space activities appeared well on its way. After the decade-long crisis of the 1990s, the country’s political leadership guided by President Putin gave new impetus to the development of national space activities and put the sector back among the top priorities of Moscow’s domestic and foreign policy agenda. Supported by the progressive recovery of Russia’s economy, renewed political stability, and an improving external environment, Russia re-asserted strong ambitions and the resolve to regain its original position on the international scene. Towards this, several major space programmes were adopted, including the Federal Space Programme 2006-2015, the Federal Target Programme on the development of Russian cosmodromes, and the Federal Target Programme on the redeployment of GLONASS. This renewed commitment to the development of space activities was duly reflected in a sharp increase in the country’s launch rate and space budget throughout the decade. Thanks to the funds made available by flourishing energy exports, Russia’s space expenditure continued to grow even in the midst of the global financial crisis. Besides new programmes and increased funding, the spectrum of activities was also widened to encompass a new focus on space applications and commercial products. -
The Orbits of Saturn's Small Satellites Derived From
The Astronomical Journal, 132:692–710, 2006 August A # 2006. The American Astronomical Society. All rights reserved. Printed in U.S.A. THE ORBITS OF SATURN’S SMALL SATELLITES DERIVED FROM COMBINED HISTORIC AND CASSINI IMAGING OBSERVATIONS J. N. Spitale CICLOPS, Space Science Institute, 4750 Walnut Street, Suite 205, Boulder, CO 80301; [email protected] R. A. Jacobson Jet Propulsion Laboratory, California Institute of Technology, 4800 Oak Grove Drive, Pasadena, CA 91109-8099 C. C. Porco CICLOPS, Space Science Institute, 4750 Walnut Street, Suite 205, Boulder, CO 80301 and W. M. Owen, Jr. Jet Propulsion Laboratory, California Institute of Technology, 4800 Oak Grove Drive, Pasadena, CA 91109-8099 Received 2006 February 28; accepted 2006 April 12 ABSTRACT We report on the orbits of the small, inner Saturnian satellites, either recovered or newly discovered in recent Cassini imaging observations. The orbits presented here reflect improvements over our previously published values in that the time base of Cassini observations has been extended, and numerical orbital integrations have been performed in those cases in which simple precessing elliptical, inclined orbit solutions were found to be inadequate. Using combined Cassini and Voyager observations, we obtain an eccentricity for Pan 7 times smaller than previously reported because of the predominance of higher quality Cassini data in the fit. The orbit of the small satellite (S/2005 S1 [Daphnis]) discovered by Cassini in the Keeler gap in the outer A ring appears to be circular and coplanar; no external perturbations are appar- ent. Refined orbits of Atlas, Prometheus, Pandora, Janus, and Epimetheus are based on Cassini , Voyager, Hubble Space Telescope, and Earth-based data and a numerical integration perturbed by all the massive satellites and each other. -
Leonid Gurvits JIVE and TU Delft June 3, 2021 ©Cristian Fattinanzi Piter Y Moons Xplorer
Leonid Gurvits JIVE and TU Delft June 3, 2021 ©Cristian Fattinanzi piter y moons xplorer Why a mission to Jupiter? Bits of history Mission challenges Where radio astronomy comes in • ~2000–2005: success of Cassini and Huygens missions (NASA, ESA, ASI) • 2006: Europlanet meeting in Berlin – “thinking aloud” on a Jovian mission • 2008: ESA-NASA jointly exploring a mission to giant planets’ satellites • ESA "Laplace" mission proposal (Blanc et al. 2009) • ESA Titan and Enceladus Mission (TandEM, Coustenis et al., 2009) • NASA Titan Explorer • 2009: two joint (ESA+NASA) concepts selected for further studies: • Europa Jupiter System Mission (EJSM) • Titan Saturn System Mission (TSSM: Coustenis et al., 2009) • 2010: EJSM-Laplace selected, consisting of two spacecraft: • ESA’s Jupiter Ganymede Orbiter (JGO) • NASA’s Jupiter Europa Orbiter (JEO) • 2012: NASA drops off; EJSM-Laplace/JGO becomes JUICE • 2013: JUICE payload selection completed by ESA; JUICE becomes an L-class mission of ESA’s Vision 2015-2025 • 2015: NASA selects Europa Clipper mission (Pappalardo et al., 2013) Voyager 1, 1979 ©NASA HOW DOES IT SEARCH FOR ORIGINS & WORK? LIFE FORMATION HABITABILITY Introduction Overarching questions JUICE JUICE Science Themes • Emergence of habitable worlds around gas giants • Jupiter system as an archetype for gas giants JUICE concept • European-led mission to the Jovian system • First orbiter of an icy moon • JGO/Laplace scenario with two Europa flybys and moderate-inclination phase at Jupiter • Science payload selected in Feb 2013, fully compatible -
HUMAN ADAPTATION to SPACEFLIGHT: the ROLE of FOOD and NUTRITION Second Edition
National Aeronautics and Human Space Administration Adaptation to Spaceflight: The Role of Food and Nutrition Second Edition Scott M. Smith Sara R. Zwart Grace L. Douglas Martina Heer National Aeronautics and Space Administration HUMAN ADAPTATION TO SPACEFLIGHT: THE ROLE OF FOOD AND NUTRITION Second Edition Scott M. Smith Grace L. Douglas Nutritionist; Advanced Food Technology Lead Scientist; Manager for Nutritional Biochemistry Manager for Exploration Food Systems Nutritional Biochemistry Laboratory Space Food Systems Laboratory Biomedical Research and Human Systems Engineering and Environmental Sciences Division Integration Division Human Health and Performance Human Health and Performance Directorate Directorate NASA Johnson Space Center NASA Johnson Space Center Houston, Texas USA Houston, Texas USA Sara R. Zwart Martina Heer Senior Scientist; Nutritionist; Deputy Manager for Nutritional Program Director Nutritional Sciences Biochemistry IU International University of Nutritional Biochemistry Laboratory Applied Sciences Biomedical Research and Bad Reichenhall, Germany Environmental Sciences Division & Human Health and Performance Adjunct Professor of Nutrition Physiology Directorate Institute of Nutritional and Food Sciences NASA Johnson Space Center University of Bonn, Germany Houston, Texas USA & Preventive Medicine and Population Health University of Texas Medical Branch Galveston, Texas USA Table of Contents Preface ......................................................................................................................... -
Janus: a Mission Concept to Explore Two NEO Binary Asteroids
Janus: A mission concept to explore two NEO Binary Asteroids D.J. Scheeres1, J.W. McMahon1, J. Hopkins2, C. Hartzell3, E.B. Bierhaus2, L.A.M. Benner4, P. Hayne1, R. Jedicke5, L. LeCorre6, S. Naidu4, P. Pravec7, and M. Ravine8 1The University of Colorado Boulder, USA; 2Lockheed Martin Inc, USA; 3University of Maryland, USA; 4Jet Propulsion Laboratory, USA; 5University of Hawaii, USA; 6Planetary Science Institute, USA; 7Astronomical Institute of the Academy of Sciences, Czech Republic; 8Malin Space Science Systems Inc, USA University of Colorado and/or Lockheed Martin Proprietary Information SIMPLEx AO: NNH17ZDA004O-SIMPLEx July 2018 Janus Mission Selected for Phase A/B! • Janus was submitted to the inaugural SIMPLEx call for proposals – Launch provided on an upcoming mission, e.g., Lucy or Psyche, for interplanetary missions – Up to $55M for a given mission • Proposals were submitted July 2018 (12 total submissions) • Announcement made last Wednesday… Janus is selected for Phase A/B! (1/3) D.J. Scheeres, A. Richard Seebass Chair, University of Colorado at Boulder !2 B-1 Use or disclosure of data contained on this sheet is subject to the restriction on the title page of this proposal University of Colorado and/or Lockheed Martin Proprietary Information University of Colorado and/or Lockheed Martin Proprietary Information SIMPLEx AO: NNH17ZDA004O-SIMPLEx July 2018 B FACT SHEET Malin SSS B-1 Use or disclosure of data contained on this sheet is subject to the restriction on the title page of this proposal University of Colorado and/or Lockheed Martin Proprietary Information University of Colorado and/or Lockheed Martin Proprietary Information SIMPLEx AO: NNH17ZDA004O-SIMPLEx July 2018 B FACT SHEET The Janus Science Objectives and corresponding Mission Implementation are focused and simple D.J. -
Into the Unknown Together the DOD, NASA, and Early Spaceflight
Frontmatter 11/23/05 10:12 AM Page i Into the Unknown Together The DOD, NASA, and Early Spaceflight MARK ERICKSON Lieutenant Colonel, USAF Air University Press Maxwell Air Force Base, Alabama September 2005 Frontmatter 11/23/05 10:12 AM Page ii Air University Library Cataloging Data Erickson, Mark, 1962- Into the unknown together : the DOD, NASA and early spaceflight / Mark Erick- son. p. ; cm. Includes bibliographical references and index. ISBN 1-58566-140-6 1. Manned space flight—Government policy—United States—History. 2. National Aeronautics and Space Administration—History. 3. Astronautics, Military—Govern- ment policy—United States. 4. United States. Air Force—History. 5. United States. Dept. of Defense—History. I. Title. 629.45'009'73––dc22 Disclaimer Opinions, conclusions, and recommendations expressed or implied within are solely those of the editor and do not necessarily represent the views of Air University, the United States Air Force, the Department of Defense, or any other US government agency. Cleared for public re- lease: distribution unlimited. Air University Press 131 West Shumacher Avenue Maxwell AFB AL 36112-6615 http://aupress.maxwell.af.mil ii Frontmatter 11/23/05 10:12 AM Page iii To Becky, Anna, and Jessica You make it all worthwhile. THIS PAGE INTENTIONALLY LEFT BLANK Frontmatter 11/23/05 10:12 AM Page v Contents Chapter Page DISCLAIMER . ii DEDICATION . iii ABOUT THE AUTHOR . ix 1 NECESSARY PRECONDITIONS . 1 Ambling toward Sputnik . 3 NASA’s Predecessor Organization and the DOD . 18 Notes . 24 2 EISENHOWER ACT I: REACTION TO SPUTNIK AND THE BIRTH OF NASA . 31 Eisenhower Attempts to Calm the Nation . -
Dynamics of Tethered Asteroid Systems to Support Planetary Defense
Eur. Phys. J. Special Topics 229, 1463{1477 (2020) c EDP Sciences, Springer-Verlag GmbH Germany, THE EUROPEAN part of Springer Nature, 2020 PHYSICAL JOURNAL https://doi.org/10.1140/epjst/e2020-900183-y SPECIAL TOPICS Regular Article Dynamics of tethered asteroid systems to support planetary defense Flaviane C.F. Venditti1;a, Luis O. Marchi2, Arun K. Misra3, Diogo M. Sanchez2, and Antonio F.B.A. Prado2 1 NASA Solar System Exploration Research Virtual Institute (SSERVI) and Planetary Radar Department, Arecibo Observatory, University of Central Florida, Arecibo, PR, USA 2 Division of Space Mechanics and Control, National Institute for Space Research, Sao Jose dos Campos, SP, Brazil 3 Mechanical Engineering Department, McGill University, Montreal, QC, Canada Received 28 August 2019 / Received in final form 23 November 2019 Published online 29 May 2020 Abstract. Every year near-Earth object (NEO) surveys discover hun- dreds of new asteroids, including the potentially hazardous asteroids (PHA). The possibility of impact with the Earth is one of the main motivations to track and study these objects. This paper presents a tether assisted methodology to deflect a PHA by connecting a smaller asteroid, altering the center of mass of the system, and consequently, moving the PHA to a safer orbit. Some of the advantages of this method are that it does not result in fragmentation, which could lead to another problem, and also the flexibility to change the configuration of the system to optimize the deflection according to the warning time. The dynamics of the PHA-tether-asteroid system is analyzed, and the amount of orbit change is determined for several initial conditions. -
The Emerging Role of Macrophages in Immune System Dysfunction Under Real and Simulated Microgravity Conditions
International Journal of Molecular Sciences Review The Emerging Role of Macrophages in Immune System Dysfunction under Real and Simulated Microgravity Conditions Yulong Sun * , Yuanyuan Kuang and Zhuo Zuo Key Laboratory for Space Biosciences & Biotechnology, Institute of Special Environmental Biophysics, School of Life Sciences, Northwestern Polytechnical University, Xi’an 710072, China; [email protected] (Y.K.); [email protected] (Z.Z.) * Correspondence: [email protected]; Tel./Fax: +86-029-88460332 Abstract: In the process of exploring space, the astronaut’s body undergoes a series of physiological changes. At the level of cellular behavior, microgravity causes significant alterations, including bone loss, muscle atrophy, and cardiovascular deconditioning. At the level of gene expression, micro- gravity changes the expression of cytokines in many physiological processes, such as cell immunity, proliferation, and differentiation. At the level of signaling pathways, the mitogen-activated protein kinase (MAPK) signaling pathway participates in microgravity-induced immune malfunction. How- ever, the mechanisms of these changes have not been fully elucidated. Recent studies suggest that the malfunction of macrophages is an important breakthrough for immune disorders in microgravity. As the first line of immune defense, macrophages play an essential role in maintaining homeostasis. They activate specific immune responses and participate in large numbers of physiological activities by presenting antigen and secreting cytokines. The purpose of this review is to summarize recent ad- Citation: Sun, Y.; Kuang, Y.; Zuo, Z. vances on the dysfunction of macrophages arisen from microgravity and to discuss the mechanisms The Emerging Role of Macrophages of these abnormal responses. Hopefully, our work will contribute not only to the future exploration in Immune System Dysfunction under Real and Simulated on the immune system in space, but also to the development of preventive and therapeutic drugs Microgravity Conditions. -
JANUS a Written Creative Work Submitted to the Faculty of San Francisco State University in Partial Fulfillment O F ^ 0 1Q the R
JANUS A Written Creative Work submitted to the faculty of San Francisco State University In partial fulfillment of ^ 0 1 q the requirements for m e w tte D q ,re e Master o f Fine Arts In Creative Writing by A i Ebashi San Francisco, California M ay 2019 Copyright by A i Ebashi 2019 CERTIFICATION OF APPROVAL I certify that I have read JANUS by Ai Ebashi, and that in my opinion this work meets the criteria for approving a thesis submitted in partial fulfillment of the requirement for the degree Master o f Fine Arts in Creative Writing at San Francisco State University. M ichelle Carter, M.F.A. Professor o f Creative Writing fry - Andrew Joron, B.A. Assistant Professor o f Creative Writing JANUS A i Ebashi San Francisco, California 2019 JANUS is a full-length play which portrays the moment of the psychic disintegration of the Roman God Janus and inner battles that ensue. It explores the themes o f gender, identity, duality, power structure and transgenderism. Through the lenses o f the divided Gods, He Janus and She Janus, who are trapped in an apocalyptic world, I used the yin and yang concept, symbol and dynamics to portray the two polarized forces that are forever opposing or conflicting but are also ultimately complementary. My approach to these themes is philosoph al but I also included physical movement, farcical element and outrageous happenings to create comedic effects. I certify that the Abstract is a correct representation o f the content o f this written creative work. -
NASA PSD Update
Lori S. Glaze, Ph.D. NASA Planetary Science Division Director 2023–2032 Planetary Science and Astrobiology Decadal Survey Venus Panel October 20, 2020 1 Lunar Discovery and Exploration 3 Artemis and PSD • Initial Commercial Lunar Payload Service (CLPS) flights will study the lunar surface and resources at the lunar poles, starting from 2021 • VIPER: Golf-cart-sized rover will investigate volatiles in lunar polar soil • Astrobotic CLPS delivery by end of 2023 • Artemis III (2024): Crew will travel to the Moon and use Human Landing System to touch down on the lunar surface • Science Definition Team (chaired by Renee Weber, MSFC, and HLS Science Lead) will define detailed science objectives • Working with ESSIO on many lunar projects, e.g., ANGSA, instrument and technology development, international collaborations 4 Mars Program 5 Mars Sample Return • New Mars Sample Return (MSR) Program, Director: Jeff Gramling • Michael Meyer is serving as Lead/Chief Scientist for MEP and MSR • NASA/ESA MOU signed on October 5th • SMD-commissioned Independent Review for MSR will provide findings in late October • Working towards two launches in 2026 (NASA lander and ESA orbiter) – on track to enter Phase A this fall • NASA/ESA MSR Sample Planning Group - Phase 2 will address science and curation planning questions • Perseverance/MSR Caching Strategy Workshop is being planned for January 2020 Future Mars activities • Mars Architecture Strategy Working Group (MASWG) established by PSD in October 2019 to: • Determine science exploration of Mars beyond MSR -
NASA's Planetary Science Portfolio (IG-20-023)
NASA Office of Inspector General National Aeronautics and Space Administration Office of Audits NASA’S PLANETARY SCIENCE PORTFOLIO September 16, 2020 Report No. IG-20-023 Office of Inspector General To report, fraud, waste, abuse, or mismanagement, contact the NASA OIG Hotline at 800-424-9183 or 800-535-8134 (TDD) or visit https://oig.nasa.gov/hotline.html. You can also write to NASA Inspector General, P.O. Box 23089, L’Enfant Plaza Station, Washington, D.C. 20026. The identity of each writer and caller can be kept confidential, upon request, to the extent permitted by law. To suggest ideas or request future audits, contact the Assistant Inspector General for Audits at https://oig.nasa.gov/aboutAll.html. RESULTS IN BRIEF NASA’s Planetary Science Portfolio NASA Office of Inspector General Office of Audits September 16, 2020 IG-20-023 (A-19-013-00) WHY WE PERFORMED THIS AUDIT NASA’s Planetary Science Division (PSD) is responsible for a portfolio of spacecraft, including orbiters, landers, rovers, and probes, that seek to advance our understanding of the solar system by exploring the Earth’s Moon, other planets and their moons, asteroids and comets, and the icy bodies beyond Pluto. Currently, the planetary science portfolio consists of 30 space flight missions in various stages of operation. PSD missions fall under three categories: Discovery (small-class missions with development costs capped at $500 million); New Frontiers (medium-class missions with estimated development costs under $1 billion); and Flagship (large-class missions costing several billion dollars). With a proposed budget averaging $2.8 billion annually for the next 5 years, PSD is forecasted to maintain the largest budget of the six divisions within NASA’s Science Mission Directorate while supporting a wide range of exploration and research activities recommended by the National Academies of Sciences, Engineering, and Medicine (National Academies) or mandated by Congress. -
Meeting Minutes
NASA ADVISORY COUNCIL PLANETARY SCIENCE ADVISORY COMMITTEE March 1-2, 2021 Virtual Meeting Washington, DC MEETING REPORT _____________________________________________________________ Amy Mainzer, Chair ____________________________________________________________ Stephen Rinehart, Executive Secretary Table of Contents Opening and Announcements, Introductions 3 PSD Status Report 3 ESSIO Update 6 Astrobiology Update 7 Planetary Defense Coordination Office 8 Mars Exploration Program/Mars Sample Return 9 Decadal Survey Update 12 Research and Analysis Program Update 15 Inclusion Diversity Equity and Accessibility (IDEA) Update 17 General Discussion 19 Analysis Group Updates MExAG 21 VEXAG 21 LEAG 21 SBAG 22 MEPAG 22 OPAG 23 MAPSIT 24 ExMAG 24 ExoPAG 24 General Discussion 25 Findings Discussion 26 Appendix A- Attendees Appendix B- Membership roster Appendix C- Agenda Appendix D- Presentations Prepared by Joan M. Zimmermann Zantech, Inc. 2 March 1, 2021 Opening and Announcements, Introductions The Executive Secretary of the Planetary Science Advisory Committee (PAC), Dr. Stephen Rinehart, opened the meeting, welcomed members of the committee, took roll, and introduced PAC Chair, Dr. Amy Mainzer. PSD Status Report Dr. Lori Glaze, Director of the Planetary Science Division (PSD), presented a status of the division, first remarking on the COVID “anniversary”—it has been one year since NASA initiated work in a virtual environment. Dr. Glaze noted that challenges associated with COVID limitations persist, especially in terms of impact to early career (EC) scientists (networking opportunities missed, potential impact of reduced levels of productivity). She noted that the impact is not uniform, and that it would behoove the community to watch where the challenges occur and react accordingly. Dr. Glaze encouraged EC scientists to reach out, contact their mentors, and try to identify gaps in order to address them.