Monovalent Cation Activation of the Radical SAM Enzyme Pyruvate Formate-Lyase Activating Enzyme
Total Page:16
File Type:pdf, Size:1020Kb
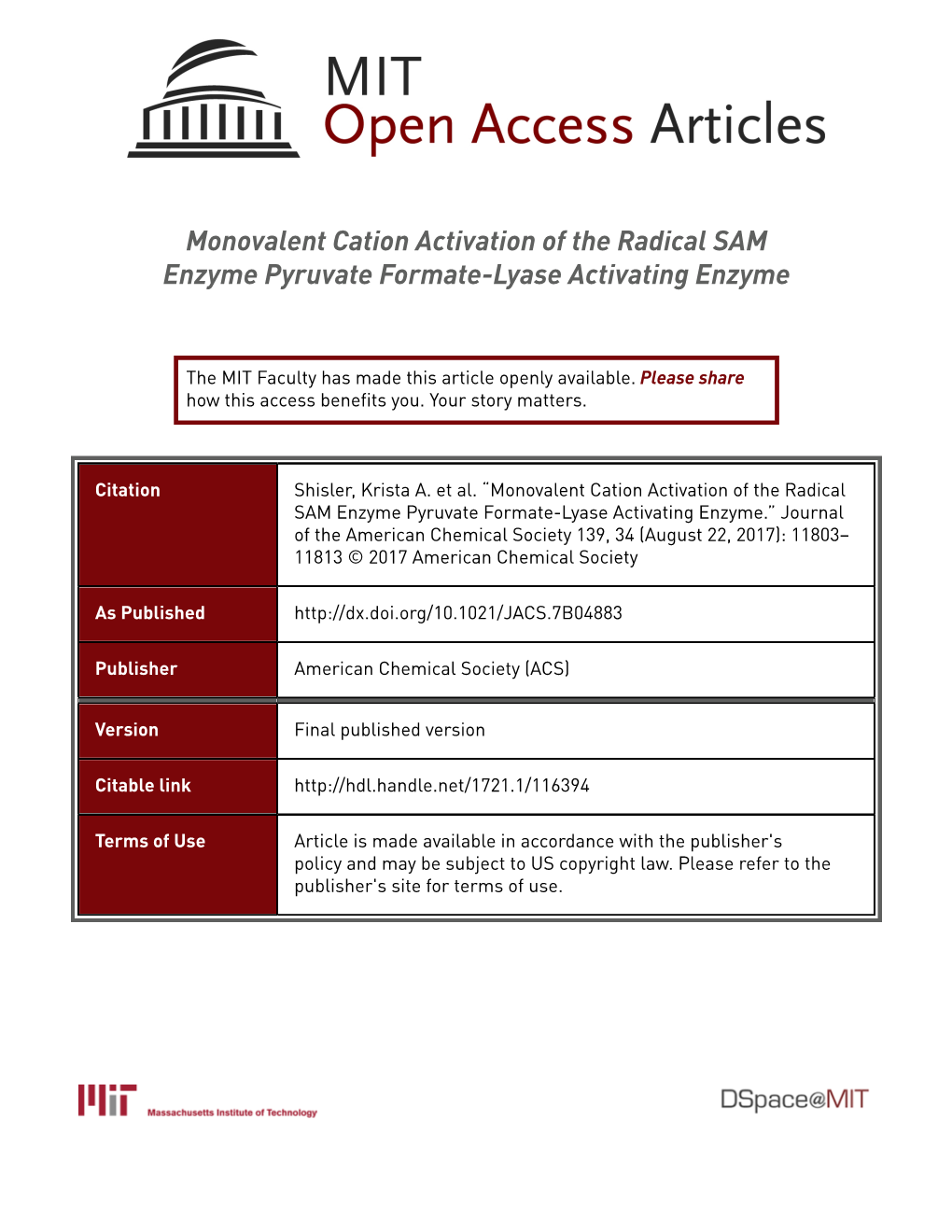
Load more
Recommended publications
-
Structure and Function of Benzylsuccinate Synthase and Related Fumarate-Adding Glycyl Radical Enzymes
Review Article J Mol Microbiol Biotechnol 2016;26:29–44 Published online: March 10, 2016 DOI: 10.1159/000441656 Structure and Function of Benzylsuccinate Synthase and Related Fumarate-Adding Glycyl Radical Enzymes a d c a Johann Heider Maciej Szaleniec Berta M. Martins Deniz Seyhan a, b e Wolfgang Buckel Bernard T. Golding a Laboratory of Microbial Biochemistry, LOEWE Center for Synthetic Microbiology, Philipps University Marburg, b c and Max-Planck-Institut für terrestrische Mikrobiologie, Marburg , and Institut für Biologie, Strukturbiologie/ d Biochemie, Humboldt-Universität zu Berlin, Berlin , Germany; Jerzy Haber Institute of Catalysis and Surface e Chemistry, Polish Academy of Sciences, Kraków , Poland; School of Chemistry, Newcastle University, Newcastle upon Tyne , UK Key Words Anaerobic Toluene Degradation Benzylsuccinate synthase · Fumarate-adding enzyme · Glycyl radical · Toluene · Alkane · Anaerobic toluene Hydrocarbons were long believed to be resistant to degradation microbial degradation in the absence of oxygen. It was therefore surprising to find that many bacteria degrade these compounds anaerobically [Aeckersberg et al., 1991; Abstract Dolfing et al., 1990; Rabus et al., 1993; Vogel and Grbic- The pathway of anaerobic toluene degradation is initiated Galic, 1986; Zeyer et al., 1986]. After the initial reports by a remarkable radical-type enantiospecific addition of the dating from 1986, the degradation pathways employed chemically inert methyl group to the double bond of a fuma- by these bacteria remained enigmatic for a decade. How- rate cosubstrate to yield (R) -benzylsuccinate as the first in- ever, over the last 20 years, various oxygen-independent termediate, as catalyzed by the glycyl radical enzyme ben- reactions have been characterized for the activation of zylsuccinate synthase. -
Abstract Gas-Phase Chemistry of Tryptophan
ABSTRACT GAS-PHASE CHEMISTRY OF TRYPTOPHAN-BASED RADICALS Andrii Piatkivskyi, Ph.D. Department of Chemistry and Biochemistry Northern Illinois University, 2014 Victor Ryzhov, Director This work is devoted to the fundamental study of gas-phase tryptophan-based radical cations. It covers: mechanisms of the radical ion formation in the gas-phase; the description and contrast of the two types of tryptophan side chain radicals (π and N-indolyl) based on their reactivities, infrared spectra, structural energetics and fragmentation patterns; investigation of the N-indolyl tryptophan radical cation fragmentation pathways; formation of the two types of tryptophan radicals (π and N-indolyl) within short peptides; their characterization and comparison based on the fragmentation patterns and reactivity; and gas-phase study of intramolecular radical migration from tryptophan to cysteine and from tryptophan to tyrosine side chains within short peptides. Two different approaches were followed to regiospecifically form desired radicals on the tryptophan side chain. The tryptophan π-radical cation was formed via electron transfer during dissociation of ternary metal complex ([CuII(terpy)(Trp)] 2+), while N-indolyl radical was formed by the homolytic cleavage of the NO group from the N-nitrosylated tryptophan during gas-phase fragmentation. Both radicals were exposed to the low-energy collision-induced dissociation in order to elucidate and contrast their fragmentation. To estimate the fragmentation pathway of the N-indolyl tryptophan radical cation, additional experiments (including H/D exchange, dissociation of tryptophan derivatives and DFT calculations) were carried out. The radical reactivity has been tested via gas-phase ion-molecule reactions with benzeneselenol, 1-propanethiol and di-tert-butyl nitroxide. -
Benzylsuccinate Synthase of Azoarcus Sp. Strain T: Cloning, Sequencing, Transcriptional Organization, and Its Role in Anaerobic Toluene and M-Xylene Mineralization
JOURNAL OF BACTERIOLOGY, Dec. 2001, p. 6763–6770 Vol. 183, No. 23 0021-9193/01/$04.00ϩ0 DOI: 10.1128/JB.183.23.6763–6770.2001 Copyright © 2001, American Society for Microbiology. All Rights Reserved. Benzylsuccinate Synthase of Azoarcus sp. Strain T: Cloning, Sequencing, Transcriptional Organization, and Its Role in Anaerobic Toluene and m-Xylene Mineralization 1 1 1,2 GYPSY R. ACHONG, ANA M. RODRIGUEZ, AND ALFRED M. SPORMANN * Environmental Engineering and Science, Department of Civil and Environmental Engineering,1 and Department of Biological Sciences,2 Stanford University, Stanford, California 94305-4020 Received 18 May 2001/Accepted 23 August 2001 Biochemical studies in Azoarcus sp. strain T have demonstrated that anaerobic oxidation of both toluene and m-xylene is initiated by addition of the aromatic hydrocarbon to fumarate, forming benzylsuccinate and 3-methyl benzylsuccinate, respectively. Partially purified benzylsuccinate synthase was previously shown to catalyze both of these addition reactions. In this study, we identified and sequenced the genes encoding benzylsuccinate synthase from Azoarcus sp. strain T and examined the role of this enzyme in both anaerobic toluene and m-xylene mineralization. Based on reverse transcription-PCR experiments and transcriptional start site mapping, we found that the structural genes encoding benzylsuccinate synthase, bssCAB, together with two additional genes, bssD and bssE, were organized in an operon in the order bssDCABE. bssD is believed to encode an activating enzyme, similar in function to pyruvate formate-lyase activase. bssE shows homology Downloaded from to tutH from Thauera aromatica strain T1, whose function is currently unknown. A second operon that is upstream of bssDCABE and divergently transcribed contains two genes, tdiS and tdiR. -
Federal University of Minas Gerais
FEDERAL UNIVERSITY OF MINAS GERAIS BIOLOGIC SCIENCES INSTITUTE BIOINFORMATIC POST-GRADUATE INTERUNIT PROGRAM Master dissertation GENOME SEQUENCING AND COMPARATIVE GENOME ANALYSIS OF Streptococcus dysgalactiae subsp. dysgalactiae, AN EMERGING PATHOGEN OF NILE TILAPIA By: Alexandra Antonieta Urrutia Zegarra Advisor: Prof. Henrique César Pereira Figueiredo, DMV, Ph.D. Belo Horizonte, MG, August of 2017 Alexandra Antonieta Urrutia Zegarra GENOME SEQUENCING AND COMPARATIVE GENOME ANALYSIS OF THE EMERGING FISH PATHOGEN Streptococcus dysgalactiae subsp. dysgalactiae Dissertation presented to the Bioinformatic Post- Graduate Interunit Program of the Federal University of Minas Gerais to obtain the title of Master in Bioinformatics. Concentration area: Genomic Bioinformatics Advisor: Prof. Henrique César Pereira Figueiredo, DMV, Ph.D. Belo Horizonte, MG, August of 2017 2 "Joq quimico tatichiy kawsay" Paul Ehrlich "Taytayta, mamayta ñañaytawan. Tioyta Jose Antonio kunan astawan qaylla ch'askakunamanta kashan.” 3 ACKNOWLEDGMENTS Thanks to the Federal University of Minas Gerais for the training offered; To the CNPq, FAPEMIG, CAPES and the Ministry of Fishery and Aquaculture for the funding of this project; To the coordination, professors and colleagues of the Post-Graduation course in Bioinformatics on the UFMG; To the members of the dissertation defense committee for accepting the invitation to evaluate this work; To my advisor Prof. Dr. Henrique César Pereira Figueiredo for the opportunity, for sharing his experience in order to improve the quality of this project and specially for letting me be part of his amazing team in AQUACEN; To the AQUACEN team for letting me feel as part of the family, to Felipe for all the teaching and all the knowledge shared; To the AQUAGIRLS for their awesome friendship, millions of laughs and incredible support; To Julio Cesar H. -
Pyruvate Formate-Lyase and a Novel Route of Eukaryotic ATP-Synthesis In
Pyruvate formate-lyase and a novel route of eukaryotic ATP-synthesis in Chlamydomonas mitochondria Ariane Atteia, Robert van Lis, Gabriel Gelius-Dietrich, Annie Adrait, Jérôme Garin, Jacques Joyard, Norbert Rolland, William Martin To cite this version: Ariane Atteia, Robert van Lis, Gabriel Gelius-Dietrich, Annie Adrait, Jérôme Garin, et al.. Pyruvate formate-lyase and a novel route of eukaryotic ATP-synthesis in Chlamydomonas mitochondria. Journal of Biological Chemistry, American Society for Biochemistry and Molecular Biology, 2006, 281, pp.9909 - 9918. 10.1074/jbc.M507862200. hal-00019406 HAL Id: hal-00019406 https://hal.archives-ouvertes.fr/hal-00019406 Submitted on 30 May 2020 HAL is a multi-disciplinary open access L’archive ouverte pluridisciplinaire HAL, est archive for the deposit and dissemination of sci- destinée au dépôt et à la diffusion de documents entific research documents, whether they are pub- scientifiques de niveau recherche, publiés ou non, lished or not. The documents may come from émanant des établissements d’enseignement et de teaching and research institutions in France or recherche français ou étrangers, des laboratoires abroad, or from public or private research centers. publics ou privés. Copyright THE JOURNAL OF BIOLOGICAL CHEMISTRY VOL. 281, NO. 15, pp. 9909–9918, April 14, 2006 © 2006 by The American Society for Biochemistry and Molecular Biology, Inc. Printed in the U.S.A. Pyruvate Formate-lyase and a Novel Route of Eukaryotic ATP Synthesis in Chlamydomonas Mitochondria*□S Received for publication, July -
PURDUE UNIVERSITY GRADUATE SCHOOL Thesis/Dissertation Acceptance
Graduate School ETD Form 9 (Revised 12/07) PURDUE UNIVERSITY GRADUATE SCHOOL Thesis/Dissertation Acceptance This is to certify that the thesis/dissertation prepared By Renae Nelson Entitled Exploring the Mechanism of Action of Spore Photoproduct Lyase Master of Science For the degree of Is approved by the final examining committee: Dr. Lei Li Chair Dr. Eric Long Dr. Michael McLeish To the best of my knowledge and as understood by the student in the Research Integrity and Copyright Disclaimer (Graduate School Form 20), this thesis/dissertation adheres to the provisions of Purdue University’s “Policy on Integrity in Research” and the use of copyrighted material. Approved by Major Professor(s): ____________________________________Dr. Lei Li ____________________________________ Approved by: Dr. Eric Long 11/20/2013 Head of the Graduate Program Date i EXPLORING THE MECHANISM OF ACTION OF SPORE PHOTOPRODUCT LYASE A Thesis Submitted to the Faculty of Purdue University by Renae Nelson In Partial Fulfillment of the Requirements for the Degree of Master of Science i December 2013 Purdue University Indianapolis, Indiana ii To Steven, thank you for holding my hand as we’ve grown up together. To my children, Adelene and Calvin, thank you for motivating me to be the best person I can be. To my Momma, for making me think that a master’s degree made you the smartest person in the world. ii iii ACKNOWLEDGEMENTS I would like to thank Dr. Lei Li for providing me with the opportunity to challenge and develop my skills as a research scientist, and his constant patience with my failures as well as my successes. -
Mapping Hole Hopping Escape Routes in Proteins
Mapping hole hopping escape routes in proteins Ruijie D. Teoa,1, Ruobing Wanga,1,2, Elizabeth R. Smithwicka, Agostino Migliorea,3, Michael J. Theriena, and David N. Beratana,b,c,3 aDepartment of Chemistry, Duke University, Durham, NC 27708; bDepartment of Biochemistry, Duke University, Durham, NC 27710; and cDepartment of Physics, Duke University, Durham, NC 27708 Edited by Michael L. Klein, Temple University, Philadelphia, PA, and approved June 25, 2019 (received for review April 13, 2019) A recently proposed oxidative damage protection mechanism in chains of 3 to 5 aromatic amino acids, with the highest occurrence proteins relies on hole hopping escape routes formed by redox- in redox proteins such as oxidoreductases and hydrolases (3). active amino acids. We present a computational tool to identify In addition to protecting redox-active sites from oxidative the dominant charge hopping pathways through these residues damage, hole hopping pathways could also act to safeguard pro- based on the mean residence times of the transferring charge teins from labilized hemes that may be produced in overoxidized along these hopping pathways. The residence times are estimated proteins like cytochrome c peroxidase (Ccp1). Labilized heme may by combining a kinetic model with well-known rate expressions be produced when H2O2 levels in Ccp1 (e.g., from Saccharomyces for the charge-transfer steps in the pathways. We identify the cerevisiae) increase ∼10-fold with yeast respiration; in this mech- most rapid hole hopping escape routes in cytochrome P450 anism, Ccp1 is activated via irreversible oxidation of the axial monooxygenase, cytochrome c peroxidase, and benzylsuccinate syn- H175 ligand to transfer its heme to apo-catalase A, after which thase (BSS). -
12) United States Patent (10
US007635572B2 (12) UnitedO States Patent (10) Patent No.: US 7,635,572 B2 Zhou et al. (45) Date of Patent: Dec. 22, 2009 (54) METHODS FOR CONDUCTING ASSAYS FOR 5,506,121 A 4/1996 Skerra et al. ENZYME ACTIVITY ON PROTEIN 5,510,270 A 4/1996 Fodor et al. MICROARRAYS 5,512,492 A 4/1996 Herron et al. 5,516,635 A 5/1996 Ekins et al. (75) Inventors: Fang X. Zhou, New Haven, CT (US); 5,532,128 A 7/1996 Eggers Barry Schweitzer, Cheshire, CT (US) 5,538,897 A 7/1996 Yates, III et al. s s 5,541,070 A 7/1996 Kauvar (73) Assignee: Life Technologies Corporation, .. S.E. al Carlsbad, CA (US) 5,585,069 A 12/1996 Zanzucchi et al. 5,585,639 A 12/1996 Dorsel et al. (*) Notice: Subject to any disclaimer, the term of this 5,593,838 A 1/1997 Zanzucchi et al. patent is extended or adjusted under 35 5,605,662 A 2f1997 Heller et al. U.S.C. 154(b) by 0 days. 5,620,850 A 4/1997 Bamdad et al. 5,624,711 A 4/1997 Sundberg et al. (21) Appl. No.: 10/865,431 5,627,369 A 5/1997 Vestal et al. 5,629,213 A 5/1997 Kornguth et al. (22) Filed: Jun. 9, 2004 (Continued) (65) Prior Publication Data FOREIGN PATENT DOCUMENTS US 2005/O118665 A1 Jun. 2, 2005 EP 596421 10, 1993 EP 0619321 12/1994 (51) Int. Cl. EP O664452 7, 1995 CI2O 1/50 (2006.01) EP O818467 1, 1998 (52) U.S. -
Radical-Sam Enzymes with Two Iron-Sulfur Clusters: Cofactor
RADICAL-SAM ENZYMES WITH TWO IRON-SULFUR CLUSTERS: COFACTOR COMPOSITION AND SPECTROSCOPIC STUDIES OF ESCHERICHIA COLI AND BACILLUS SUBTILIS BIOTIN SYNTHASE, HUMAN MOCS1A, AND THERMOTOGA MARITIMA MIAB by HEATHER LOUISE HERNÁNDEZ (Under the Direction of Michael Kenneth Johnson) ABSTRACT A new class of Fe-S proteins, termed radical-SAM enzymes, catalyzes radical reactions in a variety of biosynthetic processes. These [4Fe-4S]2+,+ cluster-containing enzymes initiate radical enzymatic reactions via reductive cleavage of S-adenosyl-L-methionine (SAM) to yield methionine and an extremely reactive 5'-deoxyadenosyl radical. A growing number of radical-SAM enzymes have recently been discovered to contain a second Fe-S cluster of unknown function, although possible roles include acting as sacrificial S-donor or anchoring and possibly activating the substrate. The combination of analytical and spectroscopic studies, including EPR, Mössbauer, UV-visible absorption/circular dichroism/variable temperature magnetic circular dichroism, and resonance Raman, have been used to investigate the cofactor composition and properties of the two cluster containing radical-SAM enzymes Escherichia coli and Bacillus subtilis biotin synthase (BioB), human MOCS1A, and Thermotoga maritima MiaB. These enzymes are involved in crucial steps in the biosynthesis of biotin and molybdopterin, and in the thiomethylation of tRNA. E. coli and B. subtilis BioB are shown to house a radical-SAM [4Fe-4S] cluster and a [2Fe-2S] cluster in separate binding sites. The function and relevance of the [2Fe-2S] cluster, which has been suggested to be the S-donor to biotin, is addressed. In E. coli BioB, the most active form of the enzyme contains a 1:1 ratio of [2Fe-2S]/[4Fe-4S] clusters and the [2Fe-2S] cluster degrades during turnover. -
New Tricks for the Glycyl Radical Enzyme Family
New tricks for the glycyl radical enzyme family The MIT Faculty has made this article openly available. Please share how this access benefits you. Your story matters. Citation Backman, Lindsey R. F. et al. “New Tricks for the Glycyl Radical Enzyme Family.” Critical Reviews in Biochemistry and Molecular Biology 52, 6 (September 2017): 674–695 © 2017 Informa UK Limited, trading as Taylor & Francis Group As Published http://dx.doi.org/10.1080/10409238.2017.1373741 Publisher Informa UK Limited Version Author's final manuscript Citable link http://hdl.handle.net/1721.1/116351 Terms of Use Creative Commons Attribution-Noncommercial-Share Alike Detailed Terms http://creativecommons.org/licenses/by-nc-sa/4.0/ HHS Public Access Author manuscript Author ManuscriptAuthor Manuscript Author Crit Rev Manuscript Author Biochem Mol Biol Manuscript Author . Author manuscript; available in PMC 2018 April 22. Published in final edited form as: Crit Rev Biochem Mol Biol. 2017 December ; 52(6): 674–695. doi:10.1080/10409238.2017.1373741. New tricks for the glycyl radical enzyme family Lindsey R.F. Backmana, Michael A. Funkd, Christopher D. Dawsonb, and Catherine. L. Drennana,b,c,* aDepartment of Chemistry, Massachusetts Institute of Technology, Cambridge, MA 02139, USA bDepartment of Biology, Massachusetts Institute of Technology, Cambridge, MA 02139, USA cHoward Hughes Medical Institute, Massachusetts Institute of Technology, Cambridge, MA 02139, USA dDepartment of Chemistry, University of Illinois at Urbana-Champaign, Urbana, IL 61801, USA Abstract Glycyl radical enzymes (GREs) are important biological catalysts in both strict and facultative anaerobes, playing key roles both in the human microbiota and in the environment. -
All Enzymes in BRENDA™ the Comprehensive Enzyme Information System
All enzymes in BRENDA™ The Comprehensive Enzyme Information System http://www.brenda-enzymes.org/index.php4?page=information/all_enzymes.php4 1.1.1.1 alcohol dehydrogenase 1.1.1.B1 D-arabitol-phosphate dehydrogenase 1.1.1.2 alcohol dehydrogenase (NADP+) 1.1.1.B3 (S)-specific secondary alcohol dehydrogenase 1.1.1.3 homoserine dehydrogenase 1.1.1.B4 (R)-specific secondary alcohol dehydrogenase 1.1.1.4 (R,R)-butanediol dehydrogenase 1.1.1.5 acetoin dehydrogenase 1.1.1.B5 NADP-retinol dehydrogenase 1.1.1.6 glycerol dehydrogenase 1.1.1.7 propanediol-phosphate dehydrogenase 1.1.1.8 glycerol-3-phosphate dehydrogenase (NAD+) 1.1.1.9 D-xylulose reductase 1.1.1.10 L-xylulose reductase 1.1.1.11 D-arabinitol 4-dehydrogenase 1.1.1.12 L-arabinitol 4-dehydrogenase 1.1.1.13 L-arabinitol 2-dehydrogenase 1.1.1.14 L-iditol 2-dehydrogenase 1.1.1.15 D-iditol 2-dehydrogenase 1.1.1.16 galactitol 2-dehydrogenase 1.1.1.17 mannitol-1-phosphate 5-dehydrogenase 1.1.1.18 inositol 2-dehydrogenase 1.1.1.19 glucuronate reductase 1.1.1.20 glucuronolactone reductase 1.1.1.21 aldehyde reductase 1.1.1.22 UDP-glucose 6-dehydrogenase 1.1.1.23 histidinol dehydrogenase 1.1.1.24 quinate dehydrogenase 1.1.1.25 shikimate dehydrogenase 1.1.1.26 glyoxylate reductase 1.1.1.27 L-lactate dehydrogenase 1.1.1.28 D-lactate dehydrogenase 1.1.1.29 glycerate dehydrogenase 1.1.1.30 3-hydroxybutyrate dehydrogenase 1.1.1.31 3-hydroxyisobutyrate dehydrogenase 1.1.1.32 mevaldate reductase 1.1.1.33 mevaldate reductase (NADPH) 1.1.1.34 hydroxymethylglutaryl-CoA reductase (NADPH) 1.1.1.35 3-hydroxyacyl-CoA -
ANAEROBIC TOLUENE DEGRADATION: GENETIC ANALYSIS of the TUT FDGH OPERON of THAUERA AROMATICA STRAIN T1 a Dissertation Presented T
ANAEROBIC TOLUENE DEGRADATION: GENETIC ANALYSIS OF THE TUT FDGH OPERON OF THAUERA AROMATICA STRAIN T1 A dissertation presented to the faculty of the College of Arts and Sciences of Ohio University In partial fulfillment of the requirements for the degree Doctor of Philosophy Reena Bhandare November 2007 2 This dissertation titled ANAEROBIC TOLUENE DEGRADATION: GENETIC ANALYSIS OF THE TUTFDGH OPERON OF THAUERA AROMATICA STRAIN T1 by REENA BHANDARE has been approved for the Department of Biological Sciences and the College of Arts and Sciences by Peter W. Coschigano Associate Professor of Microbiology Benjamin M. Ogles Dean, College of Arts and Sciences 3 ABSTRACT BHANDARE, REENA, Ph.D, November 2007, Biological Sciences ANAEROBIC TOLUENE DEGRADATION: GENETIC ANALYSIS OF THE TUTFDGH OPERON OF THAUERA AROMATICA STRAIN T1 (134 pp.) Director of Dissertation: Peter W. Coschigano Toluene is an aromatic hydrocarbon that is widely used in our everyday life. It is a major water-soluble constituent of petroleum and can pollute surface as well as ground waters. The toxic nature of toluene is responsible for causing severe health hazards. The study of toluene degrading bacteria has attracted attention because of their potential to clean up spills. Thauera aromatica strain T1 is one such bacterium capable of degrading toluene under anaerobic conditions. The tutE tutFDGH gene cluster is essential for the first step of anaerobic toluene degradation in T. aromatica strain T1. The tutF, tutD and tutG genes are proposed to code for the three subunits of the enzyme benzylsuccinate synthase, which is involved in the initial step of anaerobic toluene degradation pathway. The tutE gene is proposed to code for the enzyme benzylsuccinate synthase activase.