UNIVERSITY of CALIFORNIA RIVERSIDE Effects of in Situ
Total Page:16
File Type:pdf, Size:1020Kb
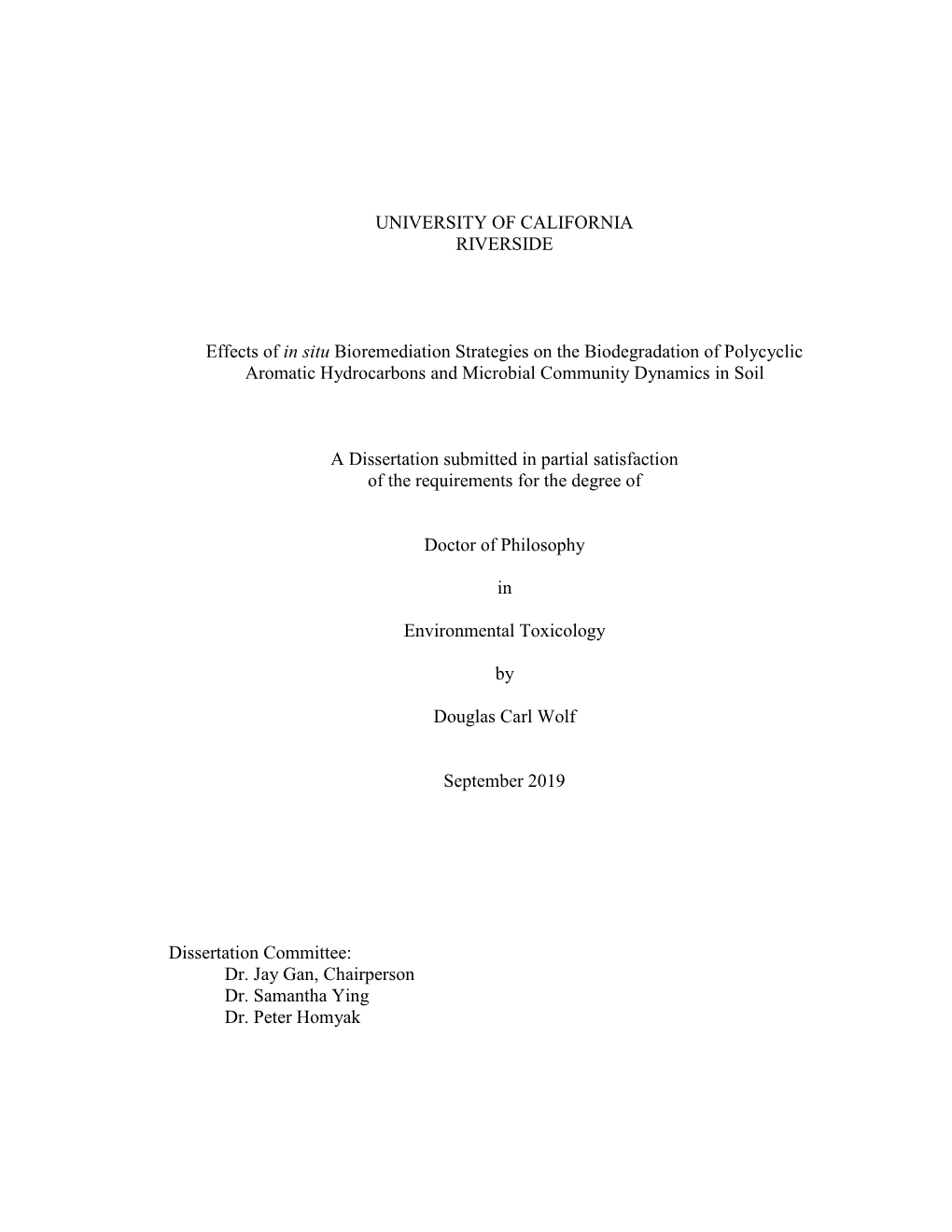
Load more
Recommended publications
-
Increased Biological Activity of Aneurinibacillus Migulanus Strains Correlates with the Production of New Gramicidin Secondary Metabolites
fmicb-08-00517 April 5, 2017 Time: 15:34 # 1 ORIGINAL RESEARCH published: 07 April 2017 doi: 10.3389/fmicb.2017.00517 Increased Biological Activity of Aneurinibacillus migulanus Strains Correlates with the Production of New Gramicidin Secondary Metabolites Faizah N. Alenezi1,2, Imen Rekik2, Ali Chenari Bouket2,3, Lenka Luptakova2,4, Hedda J. Weitz1, Mostafa E. Rateb5, Marcel Jaspars6, Stephen Woodward1 and Lassaad Belbahri2,7* 1 Institute of Biological and Environmental Sciences, University of Aberdeen, Aberdeen, UK, 2 NextBiotech, Rue Ali Edited by: Belhouane, Agareb, Tunisia, 3 Graduate School of Life and Environmental Sciences, Osaka Prefecture University, Sakai, Peter Neubauer, Japan, 4 Department of Biology and Genetics, Institute of Biology, Zoology and Radiobiology, University of Veterinary Technische Universität Berlin, Medicine and Pharmacy, Košice, Slovakia, 5 School of Science and Sport, University of the West of Scotland, Paisley, UK, Germany 6 Marine Biodiscovery Centre, Department of Chemistry, University of Aberdeen, Aberdeen, UK, 7 Laboratory of Soil Biology, Reviewed by: University of Neuchatel, Neuchatel, Switzerland Sanna Sillankorva, University of Minho, Portugal The soil-borne gram-positive bacteria Aneurinibacillus migulanus strain Nagano shows Jian Li, University of Northwestern – St. Paul, considerable potential as a biocontrol agent against plant diseases. In contrast, USA A. migulanus NCTC 7096 proved less effective for inhibition of plant pathogens. Nagano Maria Lurdes Inacio, Instituto Nacional de Investigação strain exerts biocontrol activity against some gram-positive and gram-negative bacteria, Agrária e Veterinária, Portugal fungi and oomycetes through the production of gramicidin S (GS). Apart from the *Correspondence: antibiotic effects, GS increases the rate of evaporation from the plant surface, reducing Lassaad Belbahri periods of surface wetness and thereby indirectly inhibiting spore germination. -
A Study About Protective Effect of Brevibacillus Laterosporus Texasporus Culture on Broiler Chickens Infected with Salmonella Pullorum
International Journal Of Science, Technology & Management ISSN: 2722 - 4015 A study about Protective Effect of Brevibacillus laterosporus texasporus Culture on Broiler Chickens Infected with Salmonella Pullorum Mhd Adanan Purba1,, Shoaib Ahmed Pirzado1,,Huiyi Cai1, Tesfay Hagos Haile 1 ,Aijuan Zheng1, Jiao Liu1, Jiang Chen1, Nurzainah Ginting2, Yunilas2 and Guohua Liu1* 1The Key Laboratory of Feed Biotechnology of Ministry of Agriculture, National Engineering Research Center of Biological Feed,Feed Research Institute, Chinese Academy of Agricultural Sciences, Zhongguancun Nandajie 12, Beijing, China 2Animal Production Program Study, Faculty Of Agriculture, University Of Sumatera Utara, Medan, Indonesia. *Corresponding Author: Prof. Liu Guohua Tel.:+86-1082105477, Fax number:+86-1082106077, Email : [email protected] Abstract A demand for chicken-meat is growing enormously which requires intensification in the production, so it iscrucial to improve the chicken health condition.The aim of this study is to investigate the effects of Brevibacillus laterosporus texasporus culture (BT) to the growth, immunity and blood parameters of broilers and also to determine whether the culture has a potential to act as a probiotic supplement of the fodder. A total of 300 one-day-old male Arbor Acres broilers chickens were randomly assigned to 5 treatments with 6 replications (10 individualsin each replicate) i.e.the positive control (PC)which had no challenge ofSalmonella Pullorum wasadministered inthe basal diet. Meanwhile, the negative control (NC) challenged by Salmonella Pullorumper os was administered in three form of diets, and these were included inthe diet with the composition of kitasamycin for 10 mg/kg as antibiotics growth promotor, BT for 50 mg/kg, and BT for 100 mg/kg. -
Proposal for Two New Genera, Brevibacillus Gen. Nov. and Aneurinibacillus Gen
INTERNATIONAL JOURNALOF SYSTEMATIC BACTERIOLOGY,OCt. 1996, p. 939-946 Vol. 46, No. 4 0020-7713/96/$04.00+0 Copyright 0 1996, International Union of Microbiological Societies Proposal for Two New Genera, Brevibacillus gen. nov. and Aneurinibacillus gen. nov. OSAMU SHIDA,'" HIROAKI TAKAGI,' KIYOSHI KADOWAKI,l AND KAZUO KOMAGATA' Research Laboratory, Higeta Shoyu Co., Ltd., Choshi, Chiba 288, and Department of Agricultural Chemistry, Faculty of Agriculture, Tokyo University of Agriculture, Setagaya-ku, Tokyo 156, Japan 16s rRNA gene sequences of the type strains of 11 species belonging to the Bacillus brevis and Bacillus aneurinolyticus groups were determined. On the basis of the results of gene sequence analyses, these species were separated into two clusters. The B. brevis cluster included 10 species, namely, Bacillus brevis, Bacillus agri, Bacillus centrosporus, Bacillus choshinensis, Bacillus parabrevis, Bacillus reuszeri, Bacillus formosus, Bacillus borstelensis, Bacillus luterosporus, and Bacillus thermoruber. Bacillus aneurinolyticus and Bacillus migulunus belonged to the B. aneurinolyticus cluster. Moreover, the two clusters were phylogenetically distinct from other Bacillus, Amphibacillus, Sporoluctobacillus, Paenibacillus, and Alicyclobacillus species. On the basis of our data, we propose reclassification of the B. brevis cluster as Brevibacillus gen. nov. and reclassification of the B. aneurinolyticus cluster as Aneurinibacillus gen. nov. By using 16s rRNA gene sequence alignments, two specific PCR amplification primers were designed for differentiating the two new genera from each other and from other aerobic, endospore-formingorganisms. The aerobic, rod-shaped, endospore-forming genus Bacillus is a systematically diverse taxon (5).The members of this genus exhibit a wide range of DNA base compositions, and the major amino acid compositions of the cell walls of these organisms 8. -
Brevibacillus Laterosporus, a Pathogen of Invertebrates and a Broad-Spectrum Antimicrobial Species
Insects 2013, 4, 476-492; doi:10.3390/insects4030476 OPEN ACCESS insects ISSN 2075-4450 www.mdpi.com/journal/insects/ Review Brevibacillus laterosporus, a Pathogen of Invertebrates and a Broad-Spectrum Antimicrobial Species Luca Ruiu Dipartimento di Agraria, University of Sassari, Via E. De Nicola, 07100 Sassari, Italy; E-Mail: [email protected]; Tel.: +39-079-229326; Fax: +39-079-229329 Received: 18 August 2013; in revised form: 30 August 2013 / Accepted: 30 August 2013 / Published: 5 September 2013 Abstract: Brevibacillus laterosporus, a bacterium characterized by the production of a unique canoe-shaped lamellar body attached to one side of the spore, is a natural inhabitant of water, soil and insects. Its biopesticidal potential has been reported against insects in different orders including Coleoptera, Lepidoptera, Diptera and against nematodes and mollusks. In addition to its pathogenicity against invertebrates, different B. laterosporus strains show a broad-spectrum antimicrobial activity including activity against phytopathogenic bacteria and fungi. A wide variety of molecules, including proteins and antibiotics, have been associated with the observed pathogenicity and mode of action. Before being considered as a biological control agent against plant pathogens, the antifungal and antibacterial properties of certain B. laterosporus strains have found medical interest, associated with the production of antibiotics with therapeutic effects. The recent whole genome sequencing of this species revealed its potential to produce polyketides, nonribosomal peptides, and toxins. Another field of growing interest is the use of this bacterium for bioremediation of contaminated sites by exploiting its biodegradation properties. The aim of the present review is to gather and discuss all recent findings on this emerging entomopathogen, giving a wider picture of its complex and broad-spectrum biocontrol activity. -
Genome Diversity of Spore-Forming Firmicutes MICHAEL Y
Genome Diversity of Spore-Forming Firmicutes MICHAEL Y. GALPERIN National Center for Biotechnology Information, National Library of Medicine, National Institutes of Health, Bethesda, MD 20894 ABSTRACT Formation of heat-resistant endospores is a specific Vibrio subtilis (and also Vibrio bacillus), Ferdinand Cohn property of the members of the phylum Firmicutes (low-G+C assigned it to the genus Bacillus and family Bacillaceae, Gram-positive bacteria). It is found in representatives of four specifically noting the existence of heat-sensitive vegeta- different classes of Firmicutes, Bacilli, Clostridia, Erysipelotrichia, tive cells and heat-resistant endospores (see reference 1). and Negativicutes, which all encode similar sets of core sporulation fi proteins. Each of these classes also includes non-spore-forming Soon after that, Robert Koch identi ed Bacillus anthracis organisms that sometimes belong to the same genus or even as the causative agent of anthrax in cattle and the species as their spore-forming relatives. This chapter reviews the endospores as a means of the propagation of this orga- diversity of the members of phylum Firmicutes, its current taxon- nism among its hosts. In subsequent studies, the ability to omy, and the status of genome-sequencing projects for various form endospores, the specific purple staining by crystal subgroups within the phylum. It also discusses the evolution of the violet-iodine (Gram-positive staining, reflecting the pres- Firmicutes from their apparently spore-forming common ancestor ence of a thick peptidoglycan layer and the absence of and the independent loss of sporulation genes in several different lineages (staphylococci, streptococci, listeria, lactobacilli, an outer membrane), and the relatively low (typically ruminococci) in the course of their adaptation to the saprophytic less than 50%) molar fraction of guanine and cytosine lifestyle in a nutrient-rich environment. -
Brevibacillus Massiliensis Sp. Nov
Standards in Genomic Sciences (2013) 8:1-14 DOI:10.4056/sigs.3466975 Non-contiguous finished genome sequence and description of Brevibacillus massiliensis sp. nov. Perrine Hugon1†, Ajay Kumar Mishra1†, Jean-Christophe Lagier1, Thi Thien Nguyen1, Carine Couderc1, Didier Raoult1 and Pierre-Edouard Fournier1* 1Aix-Marseille Université, URMITE, Faculté de médecine, France † These two authors have equal contribution * Corresponding author: Pierre-Edouard Fournier ([email protected]) Keywords: Brevibacillus massiliensis, genome, culturomics, taxono-genomics Brevibacillus massiliensis strain phRT sp. nov. is the type strain of B. massiliensis sp. nov., a new species within the genus Brevibacillus. This strain was isolated from the fecal flora of a woman suffering from morbid obesity. B. massiliensis is a Gram-positive aerobic rod-shaped bacterium. Here we describe the features of this organism, together with the complete genome sequence and annotation. The 5,051,018 bp long genome (1 chromosome but no plasmid) contains 5,051 protein-coding and 84 RNA genes, and exhibits a G+C content of 53.1%. Introduction Brevibacillus massiliensis strain phRT (= CSUR brevis and B. centrosporus were isolated from in- P177 = DSM 25447) is the type strain of B. door dust in schools, day care centers for children massiliensis sp. nov. This bacterium is a Gram- and animal sheds [26], and fecal flora of children, positive, spore-forming, indole negative, aerobic respectively [27]. However, several Brevibacillus and motile bacillus that was isolated from the species are also frequently isolated from humans, stool of a 26-year-old woman suffering from mor- notably in nosocomial infections, causing breast bid obesity. -
Degradation of Biological Macromolecules Supports Uncultured Microbial Populations in Guaymas Basin Hydrothermal Sediments
The ISME Journal https://doi.org/10.1038/s41396-021-01026-5 ARTICLE Degradation of biological macromolecules supports uncultured microbial populations in Guaymas Basin hydrothermal sediments 1 2 1,5 3,6 Sherlynette Pérez Castro ● Mikayla A. Borton ● Kathleen Regan ● Isabella Hrabe de Angelis ● 2 4 3 1 Kelly C. Wrighton ● Andreas P. Teske ● Marc Strous ● S. Emil Ruff Received: 8 December 2020 / Revised: 26 May 2021 / Accepted: 27 May 2021 © The Author(s) 2021. This article is published with open access Abstract Hydrothermal sediments contain large numbers of uncultured heterotrophic microbial lineages. Here, we amended Guaymas Basin sediments with proteins, polysaccharides, nucleic acids or lipids under different redox conditions and cultivated heterotrophic thermophiles with the genomic potential for macromolecule degradation. We reconstructed 20 metagenome- assembled genomes (MAGs) of uncultured lineages affiliating with known archaeal and bacterial phyla, including endospore- forming Bacilli and candidate phylum Marinisomatota.OneMarinisomatota MAG had 35 different glycoside hydrolases often in multiple copies, seven extracellular CAZymes, six polysaccharide lyases, and multiple sugar transporters. This population has 1234567890();,: 1234567890();,: the potential to degrade a broad spectrum of polysaccharides including chitin, cellulose, pectin, alginate, chondroitin, and carrageenan. We also describe thermophiles affiliating with the genera Thermosyntropha, Thermovirga,andKosmotoga with the capability to make a living on nucleic acids, lipids, or multiple macromolecule classes, respectively. Several populations seemed to lack extracellular enzyme machinery and thus likely scavenged oligo- or monomers (e.g., MAGs affiliating with Archaeoglobus) or metabolic products like hydrogen (e.g., MAGs affiliating with Thermodesulfobacterium or Desulforudaceae). The growth of methanogens or the production of methane was not observed in any condition, indicating that the tested macromolecules are not degraded into substrates for methanogenesis in hydrothermal sediments. -
Metabolites from Thermophilic Bacteria I
The Journal of Antibiotics (2014) 67, 795–798 & 2014 Japan Antibiotics Research Association All rights reserved 0021-8820/14 www.nature.com/ja NOTE Metabolites from thermophilic bacteria I: N-propionylanthranilic acid, a co-metabolite of the bacillamide class antibiotics and tryptophan metabolites with herbicidal activity from Laceyella sacchari Hirofumi Akiyama1, Naoya Oku1, Hiroaki Kasai2, Yoshikazu Shizuri2, Seitaro Matsumoto3 and Yasuhiro Igarashi1 The Journal of Antibiotics (2014) 67, 795–798; doi:10.1038/ja.2014.64; published online 28 May 2014 Since the discovery of gramicidin from Brevibacillus brevis in 1940,1 Thermoflavimicrobium, Planifilum, Mechercharimyces, Shimazuella, many important drugs including antibiotics, immunosuppressants, Desmospora, Kroppenstedtia, Marininema and Melghirimyces, based antidiabetic, antiobesity and anticancer drugs have been developed on List of Prokaryotic Names with Standing in Nomenclature: from the metabolites of mesophilic soil bacteria.2 However, as a http://www.bacterio.cict.fr/classifgenerafamilies.html). only two have reflection of worldwide efforts exerted over the years, discovering new been examined as sources for drug discovery. structures is becoming more and more difficult. Because expanding As part of our program to probe the biosynthetic potential of structural diversity of our chemical reservoir is a key requirement to unexpolited bacterial taxa, we have conducted metabolome mining in the successful drug discovery research, untapped microbes are now a strain identified as Laceyella sacchari, which resulted in the isolation gathering significant attention as new biomedical resources.3,4 of two N-acylanthranillic acids (1 and 2), one of which is new to The family Thermoactinomycetaceae (phylum Firmicutes) is one of natural products, as co-metabolites of bacillamides (3 and 4)and the kinships of Bacillaceae but has long been cataloged into N-acetyltryptamine (5). -
Brewing Microbiology– Bacteria of the Genera Bacillus, Brevibacillus and Paenibacillus
Kvasny Prum. 50 64 / 2018 (2) Brewing Microbiology– Bacteria of the Genera Bacillus, Brevibacillus and Paenibacillus DOI: 10.18832/kp201813 Brewing Microbiology – Bacteria of the Genera Bacillus, Brevibacillus and Paenibacillus and Cultivation Methods for their Detection – Part 1 Mikrobiologie pivovarské výroby – bakterie rodů Bacillus, Brevibacillus a Paenibacillus a kultivační metody pro jejich detekci – 1. část Martina BROŽOVÁ, Petra KUBIZNIAKOVÁ, Dagmar MATOULKOVÁ Department of Microbiology, Research Institute of Brewing and Malting, Lípová 15, 120 44 Praha, Czech Republic Mikrobiologické oddělení, Výzkumný ústav pivovarský a sladařský, a.s., Lípová 15, 120 44 Praha e-mail: [email protected] Reviewed paper / Recenzovaný článek Brožová, M., Kubizniaková, P., Matoulková, D., 2018: Brewing microbiology – bacteria of the genera Bacillus, Brevibacillus and Paenibacillus and cultivation methods for their detection – Part 1. Kvasny Prum. 64(2): 50–57 Bacteria of the genus Bacillus are often detected in brewing operations, but they rank among less hazardous contaminants. They are not able to survive in the vegetative form in beer and spoil it. Spores of Bacillus bacteria are present especially in malt and grain adjuncts. They are able to survive wort boiling, however in the following phases they are not able to germinate because of their sensitivity to bitter hop substances and low pH of fermenting wort and fi nished beer. We provide here an overview of basic morphological and physiological properties of these bacteria and describe their importance in brewing process. Particular attention is given to species B. cereus and B. licheniformis for which has been proved the presence of the horA gene, which is associated with resistence of lactic acid bacteria to hop substances. -
Screening of Antimicrobial Spectrum of Brevibacillus Sp. Isolated from Dairy Environment
Int.J.Curr.Microbiol.App.Sci (2018) 7(5): 850-858 International Journal of Current Microbiology and Applied Sciences ISSN: 2319-7706 Volume 7 Number 05 (2018) Journal homepage: http://www.ijcmas.com Original Research Article https://doi.org/10.20546/ijcmas.2018.705.104 Screening of Antimicrobial Spectrum of Brevibacillus sp. Isolated from Dairy Environment Sandeep Kumar1*, Ebenezer Jeyakymar2, Rubina Lawrence2, Utkarsh Singh Rathore1 and Monika Mishra1 1Division of Crop Protection, Indian Institute of Pulses Research, Kalyanpur, Kanpur, UP (East), India 2Department of Industrial Microbiology, Sam Higginbottom University of Agriculture, Technology and Sciences, Allahabad, India *Corresponding author ABSTRACT Bacteriocins and Bacteriocin like Inhibitory Substance (BLIS) are ribosomal products exhibiting antimicrobial activity. The present study aimed to screen for production of antimicrobial agents from soil bacteria of dairy environment. Borer was used to dig soil K e yw or ds and collected in sterile plastic bags. Isolation was done on tyrosine agar. To exclude non Brevibacillus brevis, spore formers the soil suspension, was heated at 80ºC. The bacterial isolates were Brevicin, Bacteriocin, identified underpinning biochemical and carbohydrate utilization tests and screened for Antagonistic activity, Tyrosine agar their antibacterial activity against methicillin resistant S. aureus and E. coli by agar well diffusion method. Among forty nine isolates three isolates were identified as Brevibacillus Article Info brevis. Screening of crude brevicin obtained from cell free broth inoculated with B. brevis Accepted: exhibited a strong antagonistic activity against S. aureus but no zone of inhibition was 10 April 2018 recorded against E. coli. The spore forming bacterium, B. brevis produced narrow Available Online: spectrum antibacterial agent. -
Bioprocessing of Brevibacillus Brevis and Bacillus Polymyxa: a Potential Biocontrol Agent of Gray Mould Disease of Strawberry Fruits Wafaa M
ISSN: 2277-3754 ISO 9001:2008 Certified International Journal of Engineering and Innovative Technology (IJEIT) Volume 3, Issue 2, August 2013 Bioprocessing Of Brevibacillus Brevis and Bacillus Polymyxa: A Potential Biocontrol Agent of Gray Mould Disease of Strawberry Fruits Wafaa M. Haggag , F. Abd-El-Kareem and S.D. Abou-Hussein pathogens. It is very important therefore to isolate and Abstract- Gray mold, caused by Botrytis cinerea is one of characterize microorganisms with antagonistic activity so the important strawberry diseases that causes losses before that biological control can be put into practice (14). Most or after harvest wherever strawberry. The effects of sustainable and environmentally acceptable control may be preharvest spray with promising bacteria i.e. Bacillus brevis achieved using biocontrol agents (BCAs) (5; 6). The and Bacillus polymyxa on gray mould and quality of current situation is mainly focused on biological control strawberry fruits pre and postharvest were evaluated. In “in (BCAs).Various microbial antagonists have been vitro” assays cells and their filtrates of both isolates investigated as potential antifungal biocontrol agents of strongly inhibited B. cinerea growth. Moreover, liquid plant diseases. We isolated more some microorganisms chromatography–mass spectrometry analysis was carried from a variety of strawberry fruit included bacteria as out to identify the antifungal components of the most Bacillus brevis and Bacillus polymyxa. B. brevis has been effective culture filtrates against gray mold pathogen. shown to produce a single cyclic as decapeptide antibiotic Bacillus polymyxa and B. brevis were found to produce large amounts of peptide polymxin B and gramicidin S, gramicidins S which has been shown to be fungicidal (7). -
Downloaded Using ‘Genbank’ As 534 Source Database
bioRxiv preprint doi: https://doi.org/10.1101/2021.07.15.452460; this version posted July 15, 2021. The copyright holder for this preprint (which was not certified by peer review) is the author/funder, who has granted bioRxiv a license to display the preprint in perpetuity. It is made available under aCC-BY-NC 4.0 International license. 1 Large-scale identification of viral quorum sensing systems reveal convergent 2 evolution of density-dependent sporulation-hijacking in bacteriophages 3 4 AUTHORS 5 Charles Bernard 1,2,*, Yanyan Li 2, Philippe Lopez 1 and Eric Bapteste 1 6 7 AFFILIATIONS 8 1 Institut de Systématique, Evolution, Biodiversité (ISYEB), Sorbonne Université, CNRS, Museum 9 National d’Histoire Naturelle, EPHE, Université des Antilles, Campus Jussieu, Bâtiment A, 4eme et. 10 Pièce 429, 75005 Paris, France 11 2 Unité Molécules de Communication et Adaptation des Micro-organismes (MCAM), CNRS, 12 Museum National d’Histoire Naturelle, CP 54, 57 rue Cuvier, 75005 Paris, France 13 14 CORRESPONDING AUTHOR 15 * Correspondence to Charles Bernard (ORCID Number: 0000-0002-8354-5350); 16 Phone: +33 (01) 44 27 34 70; E-mail address: charles.bernard@cri-p aris.org 1 bioRxiv preprint doi: https://doi.org/10.1101/2021.07.15.452460; this version posted July 15, 2021. The copyright holder for this preprint (which was not certified by peer review) is the author/funder, who has granted bioRxiv a license to display the preprint in perpetuity. It is made available under aCC-BY-NC 4.0 International license. 17 ABSTRACT 18 Quorum sensing systems (QSSs) are genetic systems supporting cell-cell or bacteriophage- 19 bacteriophage communication.