In-Situ Monitoring of Xenobiotics Using Genetically Engineered Whole-Cell-Based Microbial Biosensors: Recent Advances and Outlook
Total Page:16
File Type:pdf, Size:1020Kb
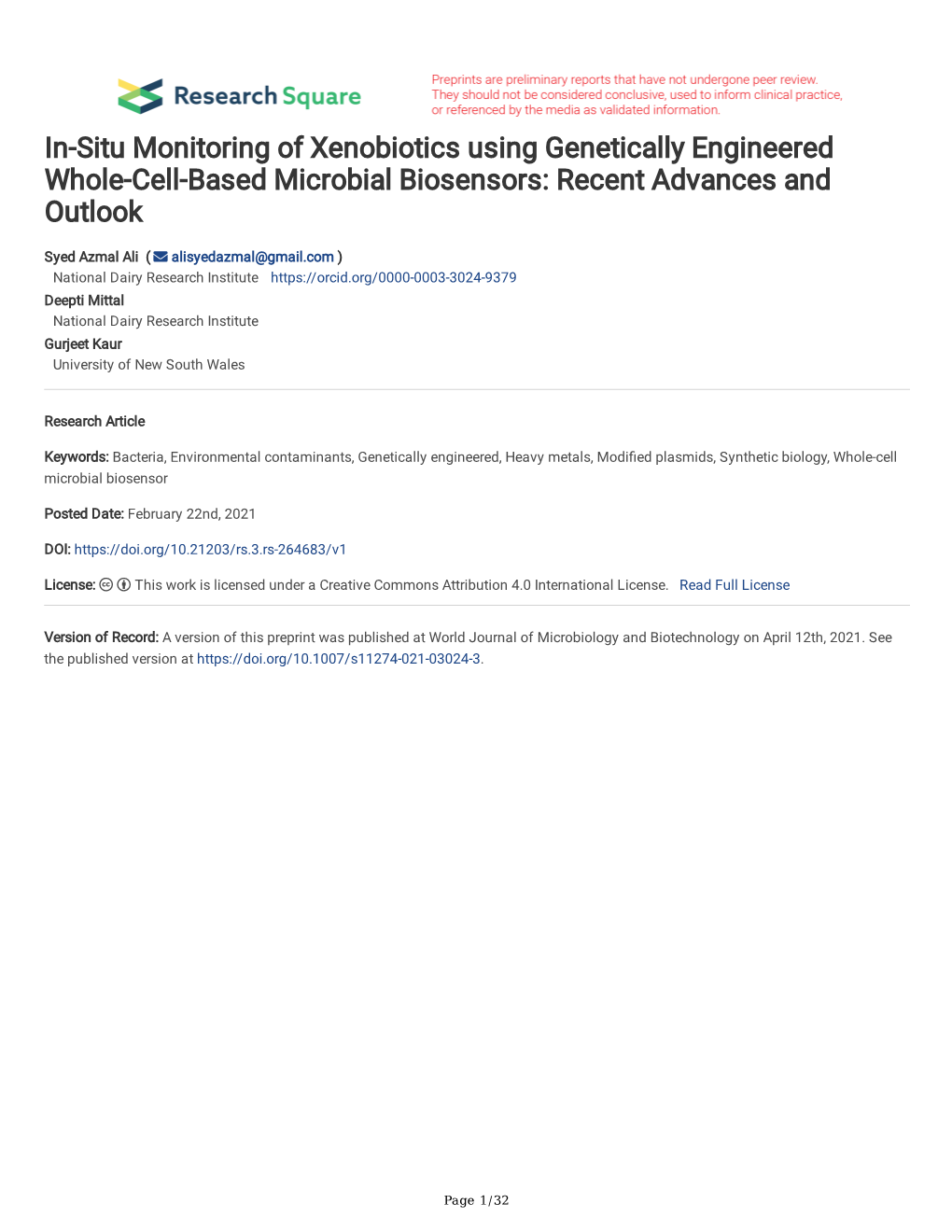
Load more
Recommended publications
-
Functional Aspects and Genomic Analysis
Discussion IV./ Discussion IV.1. The discovery of UEV protein and its role in different cellular processes In our studies of cell differentiation and cell cycle control, we have isolated a new gene that is downregulated upon cell differentiation. We have demonstrated that this gene, previously called CROC1 and considered a transcriptional activator of c-fos (Rothofsky and Lin, 1997), is highly conserved in phylogeny, and constitutes, by sequence relationship, a novel subfamily of the ubiquitin-conjugating, or E2, enzymes. We have demonstrated that these proteins are very conserved in all eukaryotic organisms and that they are very similar to the E2 enzymes in sequence and structure, but these proteins lack a conserved cysteine residue responsible for the catalytic activity of the E2 enzymes (Chen et al., 1993; Jentsch, 1992a). We have given these proteins the name UEV (ubiquitin-conjugating E2 enzyme variant). Work by other laboratories has shown that experimental mutagenesis of this cysteine in the catalytic center leads to the inactivation of the E2 enzyme activity, and the mutated protein can behave as a dominant negative variant (Banerjee et al., 1995; Sung et al., 1990; Madura et al., 1993). However, in our initial experiments with recombinant proteins, UEV did not behave as a negative regulator of ubiquitination (Sancho et al., 1998). We have demonstrated also the existence of at least two different human UEV genes, one coding for UEV1/CROC-1, and the other coding for UEV2. The second protein has also been given different names by others, DDVit-1 (Fritsche et al., 1997). 30 Discussion The transcripts from the two human UEV genes differ in their 3’untranslated regions, and produce almost identical proteins. -
Gene Prediction Using Deep Learning
FACULDADE DE ENGENHARIA DA UNIVERSIDADE DO PORTO Gene prediction using Deep Learning Pedro Vieira Lamares Martins Mestrado Integrado em Engenharia Informática e Computação Supervisor: Rui Camacho (FEUP) Second Supervisor: Nuno Fonseca (EBI-Cambridge, UK) July 22, 2018 Gene prediction using Deep Learning Pedro Vieira Lamares Martins Mestrado Integrado em Engenharia Informática e Computação Approved in oral examination by the committee: Chair: Doctor Jorge Alves da Silva External Examiner: Doctor Carlos Manuel Abreu Gomes Ferreira Supervisor: Doctor Rui Carlos Camacho de Sousa Ferreira da Silva July 22, 2018 Abstract Every living being has in their cells complex molecules called Deoxyribonucleic Acid (or DNA) which are responsible for all their biological features. This DNA molecule is condensed into larger structures called chromosomes, which all together compose the individual’s genome. Genes are size varying DNA sequences which contain a code that are often used to synthesize proteins. Proteins are very large molecules which have a multitude of purposes within the individual’s body. Only a very small portion of the DNA has gene sequences. There is no accurate number on the total number of genes that exist in the human genome, but current estimations place that number between 20000 and 25000. Ever since the entire human genome has been sequenced, there has been an effort to consistently try to identify the gene sequences. The number was initially thought to be much higher, but it has since been furthered down following improvements in gene finding techniques. Computational prediction of genes is among these improvements, and is nowadays an area of deep interest in bioinformatics as new tools focused on the theme are developed. -
Complete Genome Sequence of the Hyperthermophilic Bacteria- Thermotoga Sp
COMPLETE GENOME SEQUENCE OF THE HYPERTHERMOPHILIC BACTERIA- THERMOTOGA SP. STRAIN RQ7 Rutika Puranik A Thesis Submitted to the Graduate College of Bowling Green State University in partial fulfillment of the requirements for the degree of MASTER OF SCIENCE May 2015 Committee: Zhaohui Xu, Advisor Scott Rogers George Bullerjahn © 2015 Rutika Puranik All Rights Reserved iii ABSTRACT Zhaohui Xu, Advisor The genus Thermotoga is one of the deep-rooted genus in the phylogenetic tree of life and has been studied for its thermostable enzymes and the property of hydrogen production at higher temperatures. The current study focuses on the complete genome sequencing of T. sp. strain RQ7 to understand and identify the conserved as well as variable properties between the strains and its genus with the approach of comparative genomics. A pipeline was developed to assemble the complete genome based on the next generation sequencing (NGS) data. The pipeline successfully combined computational approaches with wet lab experiments to deliver a completed genome of T. sp. strain RQ7 that has the genome size of 1,851,618 bp with a GC content of 47.1%. The genome is submitted to Genbank with accession CP07633. Comparative genomic analysis of this genome with three other strains of Thermotoga, helped identifying putative natural transformation and competence protein coding genes in addition to the absence of TneDI restriction- modification system in T. sp. strain RQ7. Genome analysis also assisted in recognizing the unique genes in T. sp. strain RQ7 and CRISPR/Cas system. This strain has 8 CRISPR loci and an array of Cas coding genes in the entire genome. -
Cep-2020-00633.Pdf
Clin Exp Pediatr Vol. 64, No. 5, 208–222, 2021 Review article CEP https://doi.org/10.3345/cep.2020.00633 Understanding the genetics of systemic lupus erythematosus using Bayesian statistics and gene network analysis Seoung Wan Nam, MD, PhD1,*, Kwang Seob Lee, MD2,*, Jae Won Yang, MD, PhD3,*, Younhee Ko, PhD4, Michael Eisenhut, MD, FRCP, FRCPCH, DTM&H5, Keum Hwa Lee, MD, MS6,7,8, Jae Il Shin, MD, PhD6,7,8, Andreas Kronbichler, MD, PhD9 1Department of Rheumatology, Wonju Severance Christian Hospital, Yonsei University Wonju College of Medicine, Wonju, Korea; 2Severance Hospital, Yonsei University College of Medicine, Seoul, Korea; 3Department of Nephrology, Yonsei University Wonju College of Medicine, Wonju, Korea; 4Division of Biomedical Engineering, Hankuk University of Foreign Studies, Yongin, Korea; 5Department of Pediatrics, Luton & Dunstable University Hospital NHS Foundation Trust, Luton, UK; 6Department of Pediatrics, Yonsei University College of Medicine, Seoul, Korea; 7Division of Pediatric Nephrology, Severance Children’s Hospital, Seoul, Korea; 8Institute of Kidney Disease Research, Yonsei University College of Medicine, Seoul, Korea; 9Department of Internal Medicine IV (Nephrology and Hypertension), Medical University Innsbruck, Innsbruck, Austria 1,3) The publication of genetic epidemiology meta-analyses has analyses have redundant duplicate topics and many errors. increased rapidly, but it has been suggested that many of the Although there has been an impressive increase in meta-analyses statistically significant results are false positive. In addition, from China, particularly those on genetic associa tions, most most such meta-analyses have been redundant, duplicate, and claimed candidate gene associations are likely false-positives, erroneous, leading to research waste. In addition, since most suggesting an urgent global need to incorporate genome-wide claimed candidate gene associations were false-positives, cor- data and state-of-the art statistical inferences to avoid a flood of rectly interpreting the published results is important. -
Bioinformatics: a Practical Guide to the Analysis of Genes and Proteins, Second Edition Andreas D
BIOINFORMATICS A Practical Guide to the Analysis of Genes and Proteins SECOND EDITION Andreas D. Baxevanis Genome Technology Branch National Human Genome Research Institute National Institutes of Health Bethesda, Maryland USA B. F. Francis Ouellette Centre for Molecular Medicine and Therapeutics Children’s and Women’s Health Centre of British Columbia University of British Columbia Vancouver, British Columbia Canada A JOHN WILEY & SONS, INC., PUBLICATION New York • Chichester • Weinheim • Brisbane • Singapore • Toronto BIOINFORMATICS SECOND EDITION METHODS OF BIOCHEMICAL ANALYSIS Volume 43 BIOINFORMATICS A Practical Guide to the Analysis of Genes and Proteins SECOND EDITION Andreas D. Baxevanis Genome Technology Branch National Human Genome Research Institute National Institutes of Health Bethesda, Maryland USA B. F. Francis Ouellette Centre for Molecular Medicine and Therapeutics Children’s and Women’s Health Centre of British Columbia University of British Columbia Vancouver, British Columbia Canada A JOHN WILEY & SONS, INC., PUBLICATION New York • Chichester • Weinheim • Brisbane • Singapore • Toronto Designations used by companies to distinguish their products are often claimed as trademarks. In all instances where John Wiley & Sons, Inc., is aware of a claim, the product names appear in initial capital or ALL CAPITAL LETTERS. Readers, however, should contact the appropriate companies for more complete information regarding trademarks and registration. Copyright ᭧ 2001 by John Wiley & Sons, Inc. All rights reserved. No part of this publication may be reproduced, stored in a retrieval system or transmitted in any form or by any means, electronic or mechanical, including uploading, downloading, printing, decompiling, recording or otherwise, except as permitted under Sections 107 or 108 of the 1976 United States Copyright Act, without the prior written permission of the Publisher. -
Genes & Gene Finding
Genes & Gene Finding Ben Langmead Department of Computer Science Please sign guestbook (www.langmead-lab.org/teaching-materials) to tell me briefly how you are using the slides. For original Keynote files, email me ([email protected]). Gene finding Recall the "Central Dogma" and the centrality of genes DNA Transcription RNA Translation DNA molecules contain information about Protein how to create proteins; this is transcribed into RNA molecules, which, in turn, direct chemical machinery to translate the message into a protein. Hunter, Lawrence. "Life and its molecules: A brief introduction." AI Magazine 25.1 (2004): 9. Picture from: Roy H, Ibba M. Molecular biology: sticky end in protein synthesis. Nature. 2006 Sep 7;443(7107):41-2. Bacterial genes Genes (colored arrows) packed tightly into the Staphylococcus aureus genome In bacteria, one gene corresponds to one continuous interval on the genome We have good methods for predicting where these genes are Bacterial gene finding Approaches can identify exact bacterial genes with as high as 92% accuracy; can identity gene ends with ≥98% accuracy Delcher, Arthur L., et al. "Identifying bacterial genes and endosymbiont DNA with Glimmer." Bioinformatics 23.6 (2007): 673-679. Eukaryotic genes Eukaryotic genes are more complex than prokaryotic (bacterial) genes for several reasons, as we’ll see Likewise, finding eukaryotic genes computationally is harder Gene finding During the Human Genome Project, there was public debate about how many genes were in the human genome A range of predictions were -
Computational Gene Finding
Computational gene finding Devika Subramanian Comp 470 Outline (3 lectures) The biological context Lec 1 Markov models and Hidden Markov models Lec 2 Ab-initio methods for gene finding Comparative methods for gene finding Lec 3 Evaluating gene finding programs (c) Devika Subramanian, 2009 2 The biological context Introduction to the human genome and genes The central dogma: transcription and translation (c) Devika Subramanian, 2009 3 Facts about the human genome The human genome contains 3 billion chemical nucleotide bases (A, C, T, and G). About 20,500 genes are estimated to be in the human genome. Chromosome 1 (the largest human chromosome) has the most genes (2968), and the Y chromosome has the fewest (231). http://www.pnas.org/content/104/49/19428.abstract ~17,000 genes confirmed experimentally/Ensembl2009 (c) Devika Subramanian, 2009 4 More facts The average gene consists of 3000 bases, but sizes vary greatly, with the largest known human gene being dystrophin at 2.4 million bases. (c) Devika Subramanian, 2009 5 More facts Genes appear to be concentrated in random areas along the genome, with vast expanses of non-coding DNA between. About 2% of the genome encodes instructions for the synthesis of proteins. We do not know the function of more than 50% of the discovered genes. (c) Devika Subramanian, 2009 6 More facts The human genome sequence is almost (99.9%) exactly the same in all people. There are about 3 million locations where single-base DNA differences occur in humans (Single Nucleotide Polymorphisms or SNPs). Over 40% of the predicted human proteins share similarity with fruit-fly or worm proteins. -
Scheme and Syllabus of B. Tech. Biotechnology (BT) Batch 2011 Onwards
B. Tech. Biotechnology Batch- 2011 onwards PUNJAB TECHNICAL UNIVERSITY KAPURTHALA Scheme and Syllabus of B. Tech. Biotechnology (BT) Batch 2011 onwards By Board of Studies Biotechnology / Biomedical Engineering 1 | P a g e B. Tech. Biotechnology Batch- 2011 onwards Third Semester Contact Hours: 31 Hrs. Course Code Course Name Load Allocation Marks Distribution Total Credits Marks L T P Internal External BTBT301* Mathematics 4 1 - 40 60 100 5 BTBT302 Foundations of Biotechnology 4 - - 40 60 100 3 BTBT303 Microbiology 4 - - 40 60 100 3 BTBT304 Biochemistry 4 - - 40 60 100 3 BTBT305 Transport Phenomenon 4 1 - 40 60 100 5 BTBT 306 Biotech Lab –I - - 3 30 20 50 2 BTBT 307 Biotech Lab –II - - 3 30 20 50 2 BTBT308 Biotech Lab –III - - 3 30 20 50 2 BTBT309 Term Paper –I 50 - 50 1 BTCE-310 Training of 4 weeks duration after 2nd semester 60 40 100 1 Total 20 02 09 350 400 800 27 * This subject shallCccCaCarpentry, be taught by the Electrical, faculty of Plumbing, Mathematics Masonry, Department CAD - Fourth Semester Contact Hours: 31 Hrs. - Course Code Course Name - Load Allocation Marks Distribution Total Credits Marks L T P Internal External BTBT401 Biostatistics 4 1 - 40 60 100 5 BTBT402 Industrial Microbiology 4 - - 40 60 100 3 BTBT403 Immunology and 4 - - 40 60 100 4 Immunotechnology –I BTBT404 Cell & Molecular Biology 4 - - 40 60 100 3 BTBT405 Intellectual Proprietary Rights 4 - - 40 60 100 5 Bioethics and Biosafety BTBT 406 Biotech Lab –IV - - 3 30 20 50 2 BTBT 407 Biotech Lab –V - - 3 30 20 50 2 BTBT408 Biotech Lab –VI - - 3 30 20 50 2 -
Basics of Molecular Biology
Basics of Molecular Biology Martin Tompa Department of Computer Science and Engineering Department of Genome Sciences University of Washington Seattle, WA 98195-2350 U.S.A. July 6, 2003 Updated December 18, 2009 We begin with a review of the basic molecules responsible for the functioning of all organisms’ cells. Much of the material here comes from the introductory textbooks by Drlica [4], Lewin [7], and Watson et al. [10]. Good short primers have been written by Hunter [6] and Brazma¯ et al. [2]. What sorts of molecules perform the required functions of the cells of organisms? Cells have a basic tension in the roles they need those molecules to fulfill: 1. The molecules must perform the wide variety of chemical reactions necessary for life. To perform these reac- tions, cells need diverse three-dimensional structures of interacting molecules. 2. The molecules must pass on the instructions for creating their constituent components to their descendents. For this purpose, a simple one-dimensional information storage medium is the most effective. We will see that proteins provide the three-dimensional diversity required by the first role, and DNA provides the one-dimensional information storage required by the second. Another cellular molecule, RNA, is an intermediary between DNA and proteins, and plays some of each of these two roles. 1 Proteins Proteins have a variety of roles that they must fulfill: 1. They are the enzymes that rearrange chemical bonds. 2. They carry signals to and from the outside of the cell, and within the cell. 3. They transport small molecules. 4. They form many of the cellular structures. -
Computational Gene Prediction
COMPUTATIONAL GENE PREDICTION CSE/BIMM/BENG 181 MAY 24, 2011 SERGEI L KOSAKOVSKY POND [[email protected]] DEFINITIONS A gene: a nucleotide sequence that codes for a protein Gene prediction: given a genome, locate the beginning and ending position of every gene. aatgcatgcggctatgctaatgcatgcggctatgctaagctgggatccgatgacaatgcatgcggctatgctaatgcatgcg gctatgcaagctgggatccgatgactatgctaagctgggatccgatgacaatgcatgcggctatgctaatgaatggtcttgg gatttaccttggaatgctaagctgggatccgatgacaatgcatgcggctatgctaatgaatggtcttgggatttaccttgga atatgctaatgcatgcggctatgctaagctgggatccgatgacaatgcatgcggctatgctaatgcatgcggctatgcaagc tgggatccgatgactatgctaagctgcggctatgctaatgcatgcggctatgctaagctgggatccgatgacaatgcatgcg gctatgctaatgcatgcggctatgcaagctgggatcctgcggctatgctaatgaatggtcttgggatttaccttggaatgct aagctgggatccgatgacaatgcatgcggctatgctaatgaatggtcttgggatttaccttggaatatgctaatgcatgcgg ctatgctaagctgggaatgcatgcggctatgctaagctgggatccgatgacaatgcatgcggctatgctaatgcatgcggct atgcaagctgggatccgatgactatgcttaagctgcggctatgctaatgcatgcggctatgctaagctcatgcggctatgct aagctgggaatgcatgcggctatgctaagctgggatccgatgacaatgcatgcggctatgctaatgcatgcggctatgcaag ctgggatccgatgactatgctaagctgcggctatgctaatgcatgcggctatgctaagctcggctatgctaatgaatggtct tgggatttaccttggaatgctaagctgggatccgatgacaatgcatgcggctatgctaatgaatggtcttgggatttacctt ggaatatgctaatgcatgcggctatgctaagctgggaatgcatgcggctatgctaagctgggatccgatgacaatgcatgcg gctatgctaatgcatgcggctatgcaagctgggatccgatgactatgctaagctgcggctatgctaatgcatgcggctatgc taagctcatgcgg CSE/BIMM/BENG 181 MAY 24, 2011 SERGEI L KOSAKOVSKY POND [[email protected]] CENTRAL DOGMA OF MOLECULAR BIOLOGY CCTGAGCCAACTATTGATGAA -
Yersinia Pestis Genes Essential Across a Narrow Or a Broad Range of Environmental Conditions Nicola J
Senior et al. BMC Microbiology (2017) 17:163 DOI 10.1186/s12866-017-1073-8 RESEARCH ARTICLE Open Access An integrated computational-experimental approach reveals Yersinia pestis genes essential across a narrow or a broad range of environmental conditions Nicola J. Senior1, Kalesh Sasidharan2, Richard J. Saint3, Andrew E. Scott3, Mitali Sarkar-Tyson3,4, Philip M. Ireland3, Helen L Bullifent3, Z. Rong Yang1, Karen Moore1, Petra C. F. Oyston3, Timothy P. Atkins1,3, Helen S. Atkins1,3, Orkun S. Soyer2 and Richard W. Titball1* Abstract Background: The World Health Organization has categorized plague as a re-emerging disease and the potential for Yersinia pestis to also be used as a bioweapon makes the identification of new drug targets against this pathogen a priority. Environmental temperature is a key signal which regulates virulence of the bacterium. The bacterium normally grows outside the human host at 28 °C. Therefore, understanding the mechanisms that the bacterium used to adapt to a mammalian host at 37 °C is central to the development of vaccines or drugs for the prevention or treatment of human disease. Results: Using a library of over 1 million Y. pestis CO92 random mutants and transposon-directed insertion site sequencing, we identified 530 essential genes when the bacteria were cultured at 28 °C. When the library of mutants was subsequently cultured at 37 °C we identified 19 genes that were essential at 37 °C but not at 28 °C, including genes which encode proteins that play a role in enabling functioning of the type III secretion and in DNA replication and maintenance. -
Introduction to Ab Initio and Evidence-Based Gene Finding
08/09/2021 Outline Introduction to ab initio and Overview of computational gene predictions evidence-based gene finding Different types of eukaryotic gene predictors Common types of gene prediction errors Wilson Leung 08/2021 1 2 Primary goal of computational Computational gene predictions gene prediction algorithms Identify genes within genomic sequences Label each nucleotide in a genomic sequence Protein-coding genes Identify the most likely sequence of labels (i.e. optimal path) Non-coding RNA genes Regulatory regions (enhancers, promoters) Sequence TTTCACACGTAAGTATAGTGTGTGA Path 1 EEEEEEEESSIIIIIIIIIIIIIII Predictions must be confirmed experimentally Eukaryotic gene predictions have high error rates Path 2 EEEEEEEEEEEESSIIIIIIIIIII Two major types of RefSeq records: Path 3 EEEEEEEEEEEEEEEEESSIIIIII NM_/NP_ = experimentally confirmed XM_/XP_ = computational predictions Labels Exon (E) 5’ Splice Site (S) Intron (I) 3 4 Basic properties of gene prediction Prokaryotic gene predictions algorithms Prokaryotes have relatively simple gene structure Model must satisfy biological constraints Single open reading frame Coding region must begin with a start codon Alternative start codons: AUG, GUG, UUG Initial exon must occur before splice sites and introns Coding region must end with a stop codon Gene finders can predict most prokaryotic genes accurately (> 90% sensitivity and specificity) Model rules using a finite state machine (FSM) Glimmer Salzberg S., et al. Microbial gene identification using interpolated Markov models, Use species-specific characteristics to improve the NAR. (1998) 26, 544-548 accuracy of gene predictions Distribution of exon and intron sizes NCBI Prokaryotic Genome Annotation Pipeline (PGAP) Li W., et al. RefSeq: expanding the Prokaryotic Genome Annotation Pipeline reach Base frequencies (e.g., GC content, codon bias) with protein family model curation, NAR.