On the Response of a Micro Non-Destructive Testing X-Ray Detector
Total Page:16
File Type:pdf, Size:1020Kb
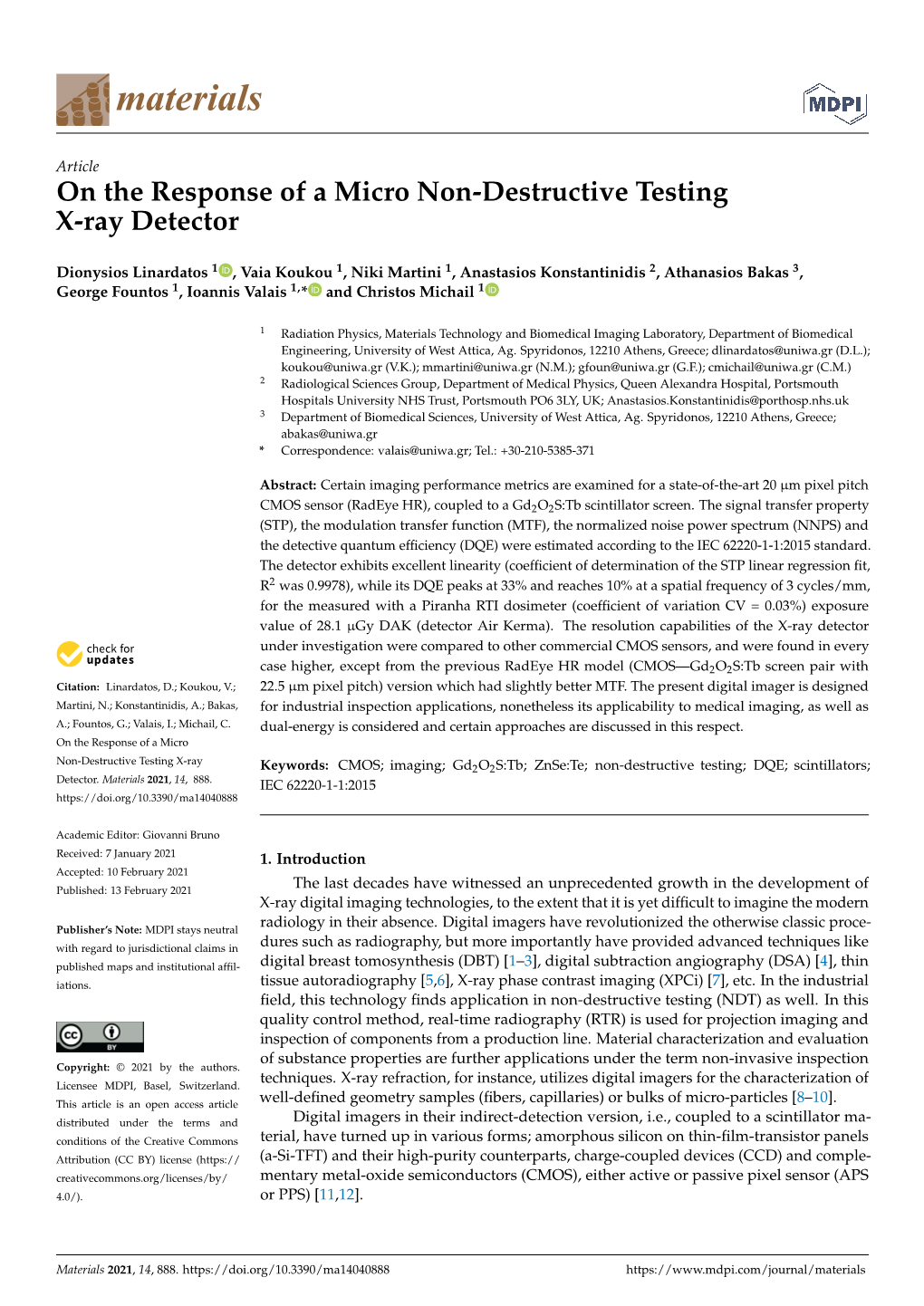
Load more
Recommended publications
-
Nanoparticles of Lanthanide and Transition Metal Oxysulfides : from Colloidal Synthesis to Structure, Surface, Optical and Magnetic Properties Clement Larquet
Nanoparticles of lanthanide and transition metal oxysulfides : from colloidal synthesis to structure, surface, optical and magnetic properties Clement Larquet To cite this version: Clement Larquet. Nanoparticles of lanthanide and transition metal oxysulfides : from colloidal synthe- sis to structure, surface, optical and magnetic properties. Material chemistry. Sorbonne Université, 2018. English. NNT : 2018SORUS432. tel-02950055 HAL Id: tel-02950055 https://tel.archives-ouvertes.fr/tel-02950055 Submitted on 27 Sep 2020 HAL is a multi-disciplinary open access L’archive ouverte pluridisciplinaire HAL, est archive for the deposit and dissemination of sci- destinée au dépôt et à la diffusion de documents entific research documents, whether they are pub- scientifiques de niveau recherche, publiés ou non, lished or not. The documents may come from émanant des établissements d’enseignement et de teaching and research institutions in France or recherche français ou étrangers, des laboratoires abroad, or from public or private research centers. publics ou privés. Sorbonne Université Ecole doctorale 397 : Physique et chimie des matériaux Laboratoire de Chimie de la Matière Condensée de Paris (LCMCP) Institut de Minéralogie, de Physique des Matériaux et de Cosmochimie (IMPMC) Nanoparticles of lanthanide and transition metal oxysulfides: from colloidal synthesis to structure, surface, optical and magnetic properties Par M. Clément Larquet Thèse de doctorat de Sorbonne Université Dirigée par Clément Sanchez et Andrea Gauzzi Présentée et soutenue publiquement le 25 septembre 2018 Devant un jury composé de : Mme. Elsje Alessandra Quadrelli Directrice de recherches - CPE Lyon Rapporteur M. Stéphane Jobic Directeur de recherches - IEMN Rapporteur Mme. Catherine Louis Directrice de recherches - SU Examinatrice Mme. Asma Tougerti Chargée de recherches – Univ. -
Synthesis and Characterization of Gd2o2s:Pr3+ Nanophosphors
Sains Malaysiana 47(8)(2018): 1861–1866 http://dx.doi.org/10.17576/jsm-2018-4708-26 3+ Synthesis and Characterization of Gd2O2S:Pr Nanophosphors using Gamma Irradiation Method 3+ (Sintesis dan Pencirian Nanofosfor Gd2O2S:Pr menggunakan Kaedah Gama Iradiasi) MUHAMMAD HASSYAKIRIN HASIM, IRMAN ABDUL RAHMAN*, SAPIZAH RAHIM, MUHAMMAD TAQIYUDDIN MAWARDI AYOB, LIYANA MOHD ALI NAPIA & SHAHIDAN RADIMAN ABSTRACT 3+ Praseodymium ion, Pr doped Gd2O2S nanophosphors were successfully synthesized via gamma irradiation route along with the heat treatment. The effect of the gamma irradiation (0-150 kGy) on the structural, morphology and 3+ photoluminescence properties of Gd2O2S:Pr were characterized via X-ray diffraction (XRD), field emission scanning electron microscope (FESEM) and photoluminescence spectroscopy (PL). The thermal properties of precursor sample were tested by the thermogravimetric analysis (TGA) and differential thermal analysis (DTA). The chemical bonding of the precursor samples were analyzed by Fourier transform infrared spectroscopy (FT-IR). The XRD result confirmed the 3+ formation of hexagonal phase of Gd2O2S:Pr without the presence of any impurities. The FESEM inspection showed the non-symmetrical shape of particles transformed into grain-like shape. The optimum photoluminescence (PL) emission 3+ 3+ intensities of Gd2-xO2S:xPr occur at 50 kGy dose of gamma irradiation and 2 mol% concentration dopant of Pr ions. 3 3 The spectra under 325 nm UV excitation shows a strong green emission at 515 nm, which match the P0 → H4 transition 3+ 3+ of Pr ions. The Gd2O2S:Pr nanophosphors possessed many useful approaches in various applications mainly as radiation detection and biomedical diagnostic. Keywords: Gadolinium oxysulfide; gamma irradiation; praseodymium doped ABSTRAK 3+ Nanofosfor Gd2O2S didop ion praseodimium, Pr berjaya disintesis melalui kaedah penyinaran gama bersama dengan rawatan haba. -
Tb3+ Phosphor Powder for X-Ray Imaging Detectors 2 2
J Nanostruct 9(4): 616-622, Autumn 2019 RESEARCH PAPER 3+ Synthesis and Characterization of Gd2O2 S: Tb Phosphor Powder for X-ray Imaging Detectors Maliheh Hassani1,*, Parvin Sarabadani2, Ali Hashemizadeh Aghda1 1 Department of Physics, Payame noor University (PNU), Tehran, Iran 2 Nuclear Science and Technology Research Institute, Karaj, Iran ARTICLE INFO ABSTRACT Gadolinium oxysulfide phosphor doped with trivalent terbium have Article History: been synthesized using urea homogenous precipitation and followed Received 11 May 2019 Accepted 11 July 2019 by sulfurization at 800 °C under argon atmosphere. Structural and Published 01 October 2019 morphological of synthesized phosphor powder were characterized by x-ray diffraction (XRD), scanning electron microscopy (SEM) and Keywords: Fourier transform infrared spectrometry (FT-IR). Hexagonal structure of 3+ Phosphor Gd2O2S:Tb phosphor was confirmed by XRD. Compositional analysis was Synthesis carried out by energy dispersive x-ray (EDX) and particle induced x-ray Terbium Doped Gadolinium emission (PIXE). Photoluminescence emission spectra was measured by Oxysulfide the fluorescent spectrometer. A sedimentation technique is used to deposit the phosphor powder directly on the glass substrate using poly vinyl X-ray Detector alcohol as a paste. A number of phosphor layers have been synthesized with the layer thickness ranging from 150 to 268 µm. Measurement results of x-ray conversion efficiency for layers were investigated using 300 kVp X-ray tube in which the maximum light output and contrast were observed for the layer with a thickness of 193 µm. Oxysulfide phosphor layer was analyzed by ion beam induced luminescence (IBIL). Emitting of green light from phosphor layer confirms its luminescence property. -
Tables of X-Ray Mass Attenuation Coefficients and Mass Energy-Absorption Coefficients 1 Kev to 20 Mev for Elements Z = 1 to 92 A
NAT'L INST. OF STAND & TECH III III III Hill HI AlllOb 243004 NIST RESEARCH INFORMATION NISTIR 5632 NIST MAY 1 6 1995 PUBLICATIONS 1 CENTER Tables of X-Ray Mass Attenuation Coefficients and Mass Energy-Absorption Coefficients 1 keV to 20 MeV for Elements Z = 1 to 92 and 48 Additional Substances of Dosimetric Interest J. H. Hubbell S. M. Seltzer U.S. DEPARTMENT OF COMMERCE Technology Administration National Institute of Standards and Technology Physics Laboratory Ionizing Radiation Division Gaithersburg, MD 20899 qo. \n> • fUlO NIST NISTIR 5632 Tables of X-Ray Mass Attenuation Coefficients and Mass Energy-Absorption Coefficients 1 keV to 20 MeV for Elements Z = 1 to 92 and 48 Additional Substances of Dosimetric Interest J. H. Hubbell S. M. Seltzer U.S. DEPARTMENT OF COMMERCE Technology Administration National Institute of Standards and Technology Physics Laboratory Ionizing Radiation Division Gaithersburg, MD 20899 May 1995 U.S. DEPARTMENT OF COMMERCE Ronald H. Brown, Secretary TECHNOLOGY ADMINISTRATION Mary L. Good, Under Secretary for Technology NATIONAL INSTITUTE OF STANDARDS AND TECHNOLOGY Arati Prabhakar, Director TABLES OF X-RAY MASS ATTENUATION COEFFICIENTS AND MASS ENERGY- ABSORPTION COEFFICIENTS 1 keV TO 20 MeV FOR ELEMENTS Z = 1 TO 92 AND 48 ADDITIONAL SUBSTANCES OF DOSIMETRIC INTEREST* + J. H. Hubbell 11830 Rocking Horse Road, Rockville, MD 20852 and S. M. Seltzer Ionizing Radiation Division, Physics Laboratory National Institute of Standards and Technology Technology Administration, U.S. Department of Commerce Gaithersburg, MD 20899 U.S. A. Abstract Tables and graphs of the photon mass attenuation coefficient p/p and the mass = 1 energy-absorption coefficient pen/p are presented for all of the elements Z to 92, and for 48 compounds and mixtures of radiological interest. -
Imaging Performance of a Cawo4/CMOS Sensor
N. Martini et alii, Frattura ed Integrità Strutturale, 50 (2019) 471-480; DOI: 10.3221/IGF-ESIS.50.39 Focused on the research activities of the Greek Society of Experimental Mechanics of Materials Imaging performance of a CaWO4/CMOS sensor Niki Martini, Vaia Koukou, George Fountos, Ioannis Valais, Ioannis Kandarakis, Christos Michail Radiation Physics, Materials Technology and Biomedical Imaging Laboratory, Department of Biomedical Engineering, University of West Attica, Athens 12210, Greece [email protected], http://orcid.org/0000-0001-5863-8013 Athanasios Bakas, Eleftherios Lavdas, Konstantinos Ninos, Georgia Oikonomou, Lida Gogou Department of Biomedical Sciences, University of West Attica, Athens 12210, Greece George Panayiotakis Department of Medical Physics, Faculty of Medicine, University of Patras, 265 00 Patras, Greece ABSTRACT. The aim of this study was to investigate the modulation transfer function (MTF) and the effective gain transfer function (eGTF) of a non- destructive testing (NDT)/industrial inspection complementary metal oxide Citation: Martini, N., Koukou, V., Fountos, semiconductor (CMOS) sensor in conjunction with a thin calcium tungstate G., Valais, I., Kandarakis, I., Michail, Ch., Ba- 2 kas, A., Lavdas, E., Ninos, K., Oikonomou, G., (CaWO4) screen. Thin screen samples, with dimensions of 2.7x3.6 cm and Gogou, L., Panayiotakis, G., Imaging perform- thickness of 118.9 μm, estimated from scanning electron microscopy-SEM im- ance of a CaWO4/CMOS sensor, Frattura ed Integrità Strutturale, 50 (2019) 471-480. ages, were extracted from an Agfa Curix universal screen and coupled to the active area of an active pixel (APS) CMOS sensor. MTF was assessed using the Received: 22.01.2019 Accepted: 22.05.2019 slanted-edge method, following the IEC 62220-1-1:2015 method. -
Development of a Focused-X-Ray Luminescence Tomography (FXLT) System
Development of a focused-X-ray luminescence tomography (FXLT) system Michael C. Lun1, Wei Zhang1, Yue Zhao1, Jeffrey Anker2, Wenxiang Cong3, Ge Wang3, Changqing Li1* 1Department of Bioengineering, School of Engineering, University of California, Merced, 5200 North Lake Road, Merced, CA 95340 2Department of Chemistry, Department of Bioengineering, Center for Optical Materials Science and Engineering Technology (COMSET), and Institute of Environment Toxicology (Cu- ENTOX), Clemson University, Clemson, SC, 29634 3Department of Biomedical Engineering, Biomedical Imaging Center, Center for Biotechnology and Interdisciplinary Studies, Rensselaer Polytechnic Institute, Troy, NY 12180 *Corresponding author: [email protected] Abstract Biophotonics is an active research area in molecular imaging, genetic diagnosis and prognosis, with direct applicability in precision medicine. However, long-standing challenges of biophotonics are well known due to low signal-to-noise ratio and poor image quality, mainly due to strong optical scattering especially in deep tissues. Recently, X-ray luminescence computed tomography (XLCT) has emerged as a hybrid molecular imaging modality and shown great promises in overcoming the optical scattering in deep tissues. However, its high spatial resolution capacity has not been fully implemented yet. In this paper, with a superfine focused X-ray beam we design a focused-X-ray luminescence tomography (FXLT) system for spatial resolution better than 150 µm and molecular sensitivity of 2 µM. First, we describe our system design. Then, we demonstrate that the molecular sensitivity of FXLT is about 5 µM considering the emitted visible photons from background. Furthermore, we analyze the measurement time per scan from measured photons numbers with a fiber bundle-PMT setup, report numerical and experimental results. -
Page References in Bold Type Refer to Primary Articles. Page References Followed by T Indicate Material in Tables. 1781
INDEX Page references in bold type refer to primary articles. Page references followed by t indicate material in tables. A Acetone [CAS: 67–64–1], 7, 899 Acid salt, 1456 A477, producing organism, 118t gas separation, 39t Acids and bases, 12–13 A42867, producing organism, 118t physical properties, 900t Acid sludge, excess air for combustion, 426t A47934, producing organism, 118t Acetone chloride, 7 Acid spoil, 1439t A51568A, producing organism, 118t Acetone cyanohydrin, 7, 465t Acid sulfite pulping process, 1379 A80407 A, producing organism, 118t Acetonephenyl-hydrazone, 7 Acid-thiol ligases, 571t A82846 A, producing organism, 118t Acetonesemicarbazone, 7 Acidulants and alkalizers (Foods), 13–14 AAAS (American Association for the Advancement Acetone-sodiumbisulfite, 7 Acifluorfen, environmental health advisories, 771t of Science), 1 Acetonitrile, 75 Acinetobacter, amphenicol susceptibility, 115t AAD-216 complex, producing organism, 118t Acetophenone, physical properties, 900t Acmite-aegerine, 14 AAD-609 complex, producing organism, 118t Acetorphan, 92t Acne vulgaris, 99 AAJ-271, producing organism, 118t Acetoxime, 7 A35512 complex, producing organism, 118t AB-65, producing organism, 118t Acetylacetone, physical properties, 900t A40926 complex, producing organism, 118t Abaca, 1, 632t Acetyl benzoyl peroxide, properties, 1237t A41030 complex, producing organism, 118t Abherent, 1 Acetyl tert-butanesulfonyl peroxide, properties, Aconitase, 282t Ablating material, 1 1237t metal chelate enzyme, 323t Ablation, 1 Acetyl chloride, physical properties, -
Predictive Formula for Electron Range Over a Large Span of Energies
Utah State University DigitalCommons@USU Posters Materials Physics Spring 4-2016 Predictive Formula for Electron Range over a Large Span of Energies Anne C. Starley Utah State University Gregory Wilson Utah State University Lisa Montierth Phillipps Utah State University JR Dennison Utah State Univesity Follow this and additional works at: https://digitalcommons.usu.edu/mp_post Part of the Condensed Matter Physics Commons Recommended Citation Anne C Starley, Greg Wilson, Lisa M Phillipps and J. R. Dennison. "Predictive Formula For Electron Range over a Large Span of Energies" 14th Spacecraft Charging Technology Conference (2016) This Conference Poster is brought to you for free and open access by the Materials Physics at DigitalCommons@USU. It has been accepted for inclusion in Posters by an authorized administrator of DigitalCommons@USU. For more information, please contact [email protected]. Predictive Formula For Electron Range over a Large Span of Energies III. Predictive Formula for NV V. Materials with Predicted Ranges Elements Organic Compound Inorganic Compound Polymer Composite 푩 Solids Actinium 13.576 Adenine 2.408 Alumina (Aluminum Oxide) 4.538 Bakelite 2.047 A-150 Tissue-Equivalent Plastic 4.562 A simple formula [Eq. (4)] was found to predict our single range parameter, Nv , as a function of only mean atomic Aluminium 5.195 Alanine 1.945 Barium Fluoride 7.125 C-552 Air-Equivalent Plastic 2.831 Adipose Tissue (ICRP) 1.554 푵 (풁풎) = 푨풁 − 푪 Americium 13.151 Cellulose Nitrate 31.722 Barium Sulfate 5.817 Cellulose Acetate Butyrate 2.059 Amber 1.462 푽 풎 Antimony 10.59 Epoxy (EP) 1.805 Beryllium oxide 3.734 Cellulose Acetate, Cellophane 2.23 Anthracene 1.616 Arsenic 8.404 Glutamine 2.064 Bismuth Germanium Oxide 7.025 Ethyl Cellulose 1.857 B-100 Bone-Equivalent Plastic 2.047 number derived from the stoichiometric formula. -
Product Brief High-Efficiency Ceramic Scintillating Crystals and Crystal Arrays
Product Brief High-efficiency Ceramic Scintillating Crystals and Crystal Arrays Highlights Description Governmental Agencies worldwide are now • Superior light output Toshiba has developed a new family of finding these systems appropriate for security, efficiency ceramic scintillators that have both high light counter-terrorism and as a means for output efficiency as well as high light detecting a variety of illegal activities including • Two to three times higher transmittance. These new ceramic the smuggling of goods and human trafficking. light output than Cadmium scintillators are ideal for use in various non- Tungstate Praseodymium doped gadolinium oxysulfide destructive industrial product inspection phosphor powder, used in scintillating screens • Fast decay times and applications, as well as in security and produced by Toshiba for many years for reduced afterglow surveillance applications to thwart terrorist medical diagnostic applications, has long threats or other illegal activities. The • Nearly transparent been recognized as a material that has a high scintillators are made of polycrystalline crystals fluorescence efficiency and short fluorescence Gadolinium Oxysulfide (GOS) crystals with decay time under x-ray excitation. More • High X-ray stopping ability dopants of either Praseodymium (Pr), recently, it was realized that this same basic Terbium (Tb) or Europium (Eu) determined material could be made into a translucent by the requirements of the application. This ceramic scintillator by a manufacturing new family of GOS crystals offers unusually technique known as Hot Isostatic Pressing high x-ray-to-light conversion efficiency with (HIP). This process, which simultaneously afterglow intensities as low as 0.1% at 3 applies high pressure uniformly from all milliseconds. -
Time-Resolved Photoluminescence and X-Ray Luminescence Studies on Rare-Earth Oxysulfide Phosphors
Time-resolved photoluminescence and X-ray luminescence studies on rare-earth oxysulfide phosphors Jonathan P. Creasey and Glenn C. Tyrrell* Applied Scintillation Technologies Ltd. Harlow, Essex, United Kingdom, CM19 5BZ ABSTRACT The use ofrare earth oxysulfide-type phosphor screens is commonplace within x-ray scanning instrumentation either in detection or imaging mode. The x-ray phosphors based on praseodymium-doped gadolinium oxysulfide (Gd2O2S:Pr) have short decay times to 10% intensity compared to the more well known europium and terbium analogues. The prompt emission of 51mm luminescence from the Pr-ion results in excellent capability for fast scanning x-ray applications in the 20-lOOps time frame. 1. INTRODUCTION X-ray phosphors with high light intensity, relatively fast decay times (<lOps) and a luminescent spectral matching to silicon photodiode arrays are increasingly becoming a necessity for scanning instrumentation for security applications. Popular phosphor hosts for these applications are the rare-earth oxysulfides, e.g. Y202S and Gd2O2S, due to the high x-ray absorption, resulting from their high atomic number (Z) and k absorption edges at 17.OkeV and 50.2keV respectively. Although much information has been published on the photoluminescence of x-ray phosphors and on the properties of these phosphor screens, very little detail on the decay and afterglow behaviour is known. Recent studies of the synchrotron luminescence ofGd2O2S:Pr revealed a fast initial decay (<1Ots) with significant afterglow [1]. In addition, a survey of Pr- doped phosphors from different manufacturers revealed a profound variation in duration, intensity and dominant visual colour ofthe afterglow. This variation ofperformance inevitably influences the reproducible production of high performance screens for sub- 1 OOis response applications. -
Tunable Magnetic Properties Of
Tunable Magnetic Properties of (Gd,Ce) 2 O 2 S Oxysulfide Nanoparticles Clément Larquet, Yannick Klein, David Hrabovsky, Andrea Gauzzi, Clément Sanchez, Sophie Carenco To cite this version: Clément Larquet, Yannick Klein, David Hrabovsky, Andrea Gauzzi, Clément Sanchez, et al.. Tunable Magnetic Properties of (Gd,Ce) 2 O 2 S Oxysulfide Nanoparticles. European Journal of Inorganic Chemistry, Wiley-VCH Verlag, 2019, 2019 (6), pp.762-765. hal-02017810 HAL Id: hal-02017810 https://hal.sorbonne-universite.fr/hal-02017810 Submitted on 13 Feb 2019 HAL is a multi-disciplinary open access L’archive ouverte pluridisciplinaire HAL, est archive for the deposit and dissemination of sci- destinée au dépôt et à la diffusion de documents entific research documents, whether they are pub- scientifiques de niveau recherche, publiés ou non, lished or not. The documents may come from émanant des établissements d’enseignement et de teaching and research institutions in France or recherche français ou étrangers, des laboratoires abroad, or from public or private research centers. publics ou privés. Tunable Magnetic Properties of (Gd,Ce)2O2S Oxysulfide Nanoparticles Clément Larquet,1,2 Yannick Klein,2 David Hrabovsky,2 Andrea Gauzzi,2 Clément Sanchez,1 Sophie Carenco1,* 1 Sorbonne Université, CNRS UMR 7574, Collège de France, Laboratoire de Chimie de la Matière Condensée de Paris (LCMCP), 4 place Jussieu, 75252 Paris Cedex 05, France 2 Institut de Minéralogie de Physique des Matériaux et de Cosmochimie (IMPMC), Sorbonne Université, CNRS UMR 7590, IRD UMR 206, MNHN, 4 place Jussieu 75252 Paris Cedex 05, France * Corresponding author e-mail: [email protected] Abstract: Nanoparticles with strong paramagnetic responses are of prime interest for advanced MRI imaging. -
The Economic Benefits of the North American Rare Earths Industry
The Economic Benefits of the North American Rare Earths Industry Economics & Statistics Department American Chemistry Council April 2014 Contents Executive Summary .................................................................................................. 1 Introduction .......................................................................................................... 2 What are Rare Earths? ............................................................................................... 2 The Rare Earth Industry ............................................................................................. 5 Upstream Rare Earth Supplier Economic Impact ................................................................ 8 Downstream Rare Earth Industry End-Use Markets Economic Impact ...................................... 10 Intermediate Products .......................................................................................... 15 Magnets & Magnetic Powders ............................................................................... 15 Catalysts ....................................................................................................... 17 Metallurgical Additives ...................................................................................... 18 Polishing Powders ............................................................................................ 19 Phosphors ...................................................................................................... 20 Glass Additives ...............................................................................................