Viruses Are Essential Agents Within the Roots and Stem of the Tree of Life Luis P
Total Page:16
File Type:pdf, Size:1020Kb
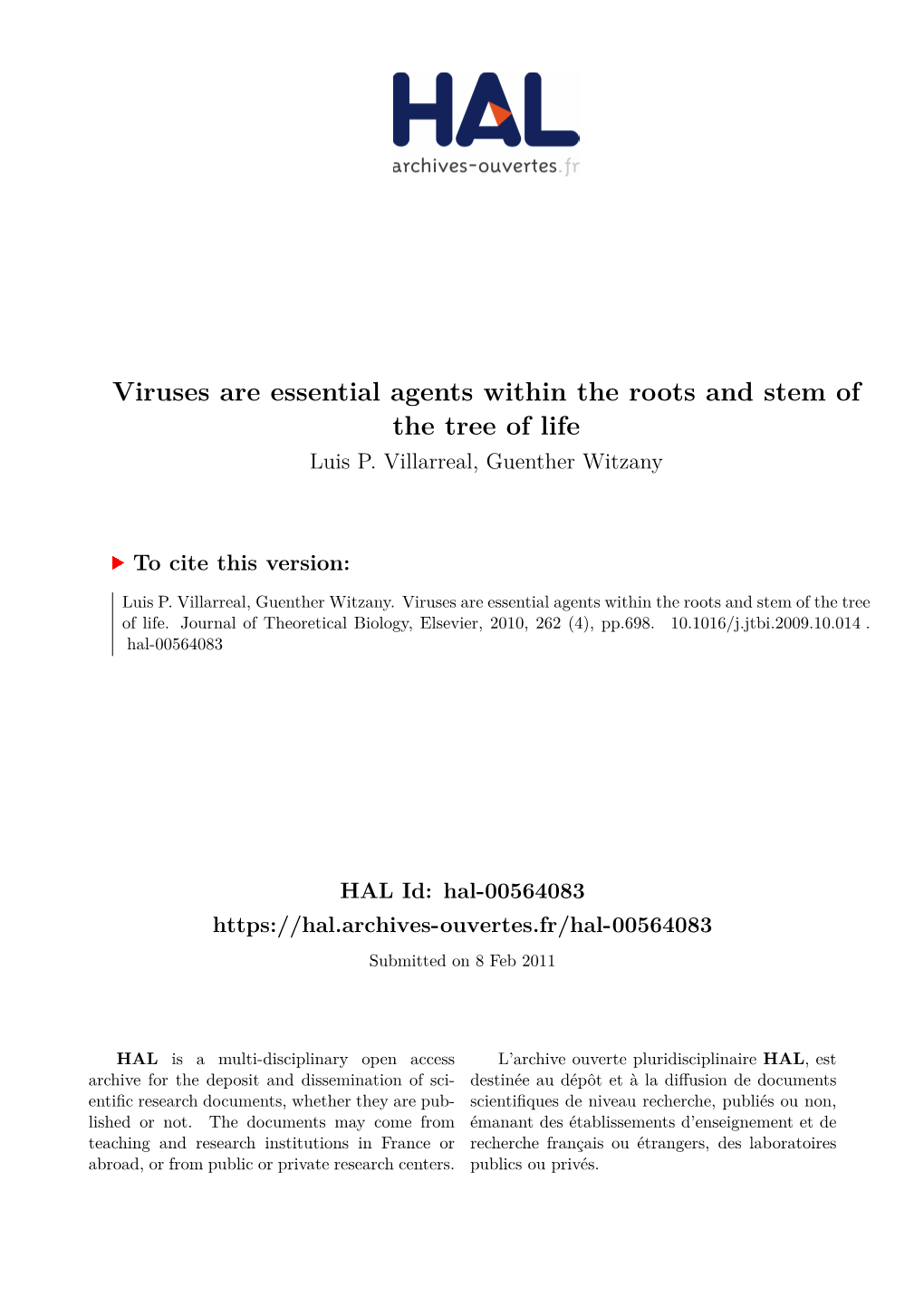
Load more
Recommended publications
-
Geve: a Genome-Based Endogenous Viral Element Database Provides
Database, 2016, 1–8 doi: 10.1093/database/baw087 Original article Original article Downloaded from https://academic.oup.com/database/article-abstract/doi/10.1093/database/baw087/2630466 by guest on 24 July 2019 gEVE: a genome-based endogenous viral element database provides comprehensive viral protein-coding sequences in mammalian genomes So Nakagawa1,2,* and Mahoko Ueda Takahashi2 1Department of Molecular Life Science, Tokai University School of Medicine, 143 Shimokasuya, Isehara, Kanagawa 259-1193, Japan and 2Micro/Nano Technology Center, Tokai University, 411 Kitakaname, Hiratsuka, Kanagawa, 259-1292, Japan *Corresponding author: Tel: þ81 463 93 1121 ext. 2661; Fax: þ81 463 93 5418; Email: [email protected] Citation details: Nakagawa,S. and Ueda Takahashi,M. gEVE: a genome-based endogenous viral element database pro- vides comprehensive viral protein-coding sequences in mammalian genomes. Database (2016) Vol. 2016: article ID baw087; doi:10.1093/database/baw087 Received 15 December 2015; Revised 15 April 2016; Accepted 4 May 2016 Abstract In mammals, approximately 10% of genome sequences correspond to endogenous viral elements (EVEs), which are derived from ancient viral infections of germ cells. Although most EVEs have been inactivated, some open reading frames (ORFs) of EVEs obtained functions in the hosts. However, EVE ORFs usually remain unannotated in the genomes, and no databases are available for EVE ORFs. To investigate the function and evolution of EVEs in mammalian genomes, we developed EVE ORF databases for 20 genomes of 19 mammalian species. A total of 736,771 non-overlapping EVE ORFs were identified and archived in a database named gEVE (http://geve.med.u-tokai.ac.jp). -
Diversity of Large DNA Viruses of Invertebrates ⇑ Trevor Williams A, Max Bergoin B, Monique M
Journal of Invertebrate Pathology 147 (2017) 4–22 Contents lists available at ScienceDirect Journal of Invertebrate Pathology journal homepage: www.elsevier.com/locate/jip Diversity of large DNA viruses of invertebrates ⇑ Trevor Williams a, Max Bergoin b, Monique M. van Oers c, a Instituto de Ecología AC, Xalapa, Veracruz 91070, Mexico b Laboratoire de Pathologie Comparée, Faculté des Sciences, Université Montpellier, Place Eugène Bataillon, 34095 Montpellier, France c Laboratory of Virology, Wageningen University, Droevendaalsesteeg 1, 6708 PB Wageningen, The Netherlands article info abstract Article history: In this review we provide an overview of the diversity of large DNA viruses known to be pathogenic for Received 22 June 2016 invertebrates. We present their taxonomical classification and describe the evolutionary relationships Revised 3 August 2016 among various groups of invertebrate-infecting viruses. We also indicate the relationships of the Accepted 4 August 2016 invertebrate viruses to viruses infecting mammals or other vertebrates. The shared characteristics of Available online 31 August 2016 the viruses within the various families are described, including the structure of the virus particle, genome properties, and gene expression strategies. Finally, we explain the transmission and mode of infection of Keywords: the most important viruses in these families and indicate, which orders of invertebrates are susceptible to Entomopoxvirus these pathogens. Iridovirus Ó Ascovirus 2016 Elsevier Inc. All rights reserved. Nudivirus Hytrosavirus Filamentous viruses of hymenopterans Mollusk-infecting herpesviruses 1. Introduction in the cytoplasm. This group comprises viruses in the families Poxviridae (subfamily Entomopoxvirinae) and Iridoviridae. The Invertebrate DNA viruses span several virus families, some of viruses in the family Ascoviridae are also discussed as part of which also include members that infect vertebrates, whereas other this group as their replication starts in the nucleus, which families are restricted to invertebrates. -
Virus World As an Evolutionary Network of Viruses and Capsidless Selfish Elements
Virus World as an Evolutionary Network of Viruses and Capsidless Selfish Elements Koonin, E. V., & Dolja, V. V. (2014). Virus World as an Evolutionary Network of Viruses and Capsidless Selfish Elements. Microbiology and Molecular Biology Reviews, 78(2), 278-303. doi:10.1128/MMBR.00049-13 10.1128/MMBR.00049-13 American Society for Microbiology Version of Record http://cdss.library.oregonstate.edu/sa-termsofuse Virus World as an Evolutionary Network of Viruses and Capsidless Selfish Elements Eugene V. Koonin,a Valerian V. Doljab National Center for Biotechnology Information, National Library of Medicine, Bethesda, Maryland, USAa; Department of Botany and Plant Pathology and Center for Genome Research and Biocomputing, Oregon State University, Corvallis, Oregon, USAb Downloaded from SUMMARY ..................................................................................................................................................278 INTRODUCTION ............................................................................................................................................278 PREVALENCE OF REPLICATION SYSTEM COMPONENTS COMPARED TO CAPSID PROTEINS AMONG VIRUS HALLMARK GENES.......................279 CLASSIFICATION OF VIRUSES BY REPLICATION-EXPRESSION STRATEGY: TYPICAL VIRUSES AND CAPSIDLESS FORMS ................................279 EVOLUTIONARY RELATIONSHIPS BETWEEN VIRUSES AND CAPSIDLESS VIRUS-LIKE GENETIC ELEMENTS ..............................................280 Capsidless Derivatives of Positive-Strand RNA Viruses....................................................................................................280 -
RNA Viruses of Amblyomma Variegatum and Rhipicephalus Microplus and Cattle Susceptibility in the French Antilles
Preprints (www.preprints.org) | NOT PEER-REVIEWED | Posted: 12 December 2019 doi:10.20944/preprints201912.0172.v1 Peer-reviewed version available at Viruses 2020, 12, 144; doi:10.3390/v12020144 Article RNA viruses of Amblyomma variegatum and Rhipicephalus microplus and cattle susceptibility in the French Antilles Mathilde Gondard 1,2,#, Sarah Temmam 3,#, Elodie Devillers 1, Valérie Pinarello 2,4, Thomas Bigot 3,5, Delphine Chrétien 3, Rosalie Aprelon 2,4, Muriel Vayssier-Taussat 1, Emmanuel Albina 2,4, Marc Eloit 3,6* and Sara Moutailler 1* 1 UMR BIPAR, Animal Health Laboratory, ANSES, INRA, Ecole Nationale Vétérinaire d’Alfort, Université Paris-Est, Maisons-Alfort, France; [email protected]; [email protected]; [email protected]; [email protected] 2 CIRAD, UMR ASTRE, F-97170 Petit-Bourg, Guadeloupe, France; [email protected]; [email protected]; [email protected] 3 Institut Pasteur, Biology of Infection Unit, Inserm U1117, Pathogen Discovery Laboratory, Paris, France; [email protected] ; [email protected] ; [email protected] ; [email protected] 4 ASTRE, Univ Montpellier, CIRAD, INRA, Montpellier, France; [email protected]; [email protected]; [email protected] 5 Institut Pasteur – Bioinformatics and Biostatistics Hub – Computational Biology Department, Institut Pasteur, USR 3756 CNRS, Paris, France. 6 National Veterinary School of Alfort, Paris-Est University, Maisons-Alfort, 94704 Cedex, France. [email protected] # These authors contributed equally. * Correspondence: [email protected]; Tel.: +33 1 49 77 46 50; [email protected]; Tel.: +33 1 44 38 92 16 Abstract: Ticks transmit a wide variety of pathogens including bacteria, parasites and viruses. -
The Origin and Evolution of Viruses
Mini Review Agri Res & Tech: Open Access J Volume 21 Issue 5 - June 2019 Copyright © All rights are reserved by Luka AO Awata DOI: 10.19080/ARTOAJ.2019.21.556181 The Central Question in Virology: The Origin and Evolution of Viruses Luka AO Awata1*, Beatrice E Ifie2, Pangirayi Tongoona2, Eric Danquah2, Samuel Offei2 and Phillip W Marchelo D’ragga3 1Directorate of Research, Ministry of Agriculture and Food Security, South Sudan 2College of Basic and Applied Sciences, University of Ghana, Ghana 3Department of Agricultural Sciences, University of Juba, South Sudan Submission: June 01, 2019; Published: June 12, 2019 *Corresponding author: Luka AO Awata, Directorate of Research, Ministry of Agriculture and Food Security, Ministries Complex, Parliament Road, P.O. Box 33, Juba, South Sudan Abstract Viruses are major threats to both animals and plants worldwide. A virus exists as a set of one or more nucleic acid molecules normally encased in a protective coat of protein or lipoprotein. It is able to replicate itself within suitable host cells, causing diseases to plants and animals. While the three domains of life trace their linages back to a single protein (the Last Universal Cellular Ancestor (LUCA), information on parental molecule from which all viruses descended is inadequate. Structural analyses of capsid proteins suggest that there is no universal viral protein and different types of virions are mostly formed independently. As a result, it is impossible to neither include viruses in the Tree of Life of LUCA nor to draw a universal tree of viruses analogous to the tree of life. Although the concepts on the origin and evolution of viruses are well documented, the structure and biological activities of viruses are paradoxical. -
XL Reunión Anual SBBMCH 26-29 De Septiembre 2017, Puerto Varas
XL Reunión Anual SBBMCH 26-29 de Septiembre 2017, Puerto Varas FALLING IN LOVE WITH SCIENCE ... A WAY OF LIFE The Chilean Society for Biochemistry and Molecular Biology promotes theoretical and experimental research, leading to the advancement and dissemination of Biochemistry and Molecular Biology in Chile. The Society also encourages and promotes initiatives aimed at maximizing the use of science for the benefit of the country and its citizens. This dream was founded in 1974, when Dr Hermann Niemeyer, Dr Marco Perreta, Dr Lylian Clark, Dr Enrique Beytia, Dr Arnaldo Foradori and Dr Arturo Yudelevich signed the document that gave legal status to the Chilean Society for Biochemistry and Molecular Biology. Subsequently, the first Annual Meeting was held in 1977. This year, our society celebrates the XL version of its Annual Meeting. Despite the passage of time, the quality, excellence and love for science remain the central axis of each and every one of our Meetings. Currently, the Society has more than 150 members, including researchers from Chilean and foreign universities. The enthusiasm and dedication to scientific activities of all our members constitute the body and soul of this Society. Our Annual Meeting aims to be an open door to the scientific community, at national and international levels, and a showcase for scientific excellence. We hope that the colleagues who entrusted us with the dissemination of their work at our meeting, can appreciate our firm commitment to high-quality research, with the solid aim of pursuing the advancement of biochemistry and molecular biology. As the Directive of the Society, we wish to transmit a strong signal that each member is important to us. -
Identification of Small Endogenous Viral Elements Within Host
IDENTIFICATION OF SMALL ENDOGENOUS VIRAL ELEMENTS WITHIN HOST GENOMES by Edward C. Davis, Jr. A thesis submitted in partial fulfillment of the requirements for the degree of Master of Science in Computer Science Boise State University May 2016 c 2016 Edward C. Davis, Jr. ALL RIGHTS RESERVED BOISE STATE UNIVERSITY GRADUATE COLLEGE DEFENSE COMMITTEE AND FINAL READING APPROVALS of the thesis submitted by Edward C. Davis, Jr. Thesis Title: Identification of Small Endogenous Viral Elements within Host Genomes Date of Final Oral Examination: 04 March 2016 The following individuals read and discussed the thesis submitted by student Edward C. Davis, Jr., and they evaluated his presentation and response to questions during the final oral examination. They found that the student passed the final oral examination. Timothy Andersen, Ph.D. Chair, Supervisory Committee Amit Jain, Ph.D. Member, Supervisory Committee Gregory Hampikian, Ph.D. Member, Supervisory Committee The final reading approval of the thesis was granted by Timothy Andersen, Ph.D., Chair, Supervisory Committee. The thesis was approved for the Graduate College by John R. Pelton, Ph.D., Dean of the Graduate College. Dedicated to Elaina, Arianna, and Zora. iv ACKNOWLEDGMENTS The author wishes to express gratitude to the members of the supervisory com- mittee for providing guidance and patience. v ABSTRACT A parallel string matching software architecture has been developed (incorpo- rating several algorithms) to identify small genetic sequences in large genomes. En- dogenous viral elements (EVEs) are sequences originating in the genomes of viruses that have become integrated into the chromosomes of sperm or egg cells of infected hosts, and passed to subsequent generations. -
A Dedicated Platform for Endogenous Viral Elements in Fishes, Amphibians
bioRxiv preprint doi: https://doi.org/10.1101/529354; this version posted January 24, 2019. The copyright holder for this preprint (which was not certified by peer review) is the author/funder, who has granted bioRxiv a license to display the preprint in perpetuity. It is made available under aCC-BY-NC 4.0 International license. FabriEVEs: A dedicated platform for endogenous viral elements in fishes, amphibians, birds, reptiles and invertebrates Jixing Zhong1,2,3§, Jiacheng Zhu2,3§, Zaoxu Xu2,3, Geng Zou4, JunWei Zhao5, SanJie Jiang2, Wei Zhang6, Jun Xia2,3,7, Lin Yang2,3, Fang Li5, Ya Gao3, Fang Chen3, Yiquan Wu8*, Dongsheng Chen3* 1 School of Biological Sciences and Medical Engineering, Southeast University, Xuanwu District, Nanjing 210096, China 2 BGI Education Center, University of Chinese Academy of Sciences,Beishan Industrial Zone,Shenzhen, 518083, China. 3 BGI-Shenzhen,Beishan Industrial Zone,Shenzhen, 518083, China. 4 State Key Laboratory of Agricultural Microbiology, College of Veterinary Medicine, Huazhong Agricultural University, Wuhan 430070, China 5 Department of Gynaecology, Shanghai First Maternity and Infant Hospital, Tongji University School of Medicine, Shanghai, 200040, China. 6 Sigmovir Biosystems Inc., 9610 Medical Center Drive, Suite 100, Rockville, MD, 20850, USA 7 MGI, BGI-Shenzhen, Beishan Industrial Zone,Shenzhen, 518083, China. 8 Current address: HIV and AIDS Malignancy Branch, Center for Cancer Research, National Cancer Institute, National Institutes of Health, Bethesda, MD 20892; [email protected] §, These authors contribute equally to this work *, Corresponding authors: Dongsheng Chen, [email protected] Yiquan Wu, [email protected] bioRxiv preprint doi: https://doi.org/10.1101/529354; this version posted January 24, 2019. -
Characterization of a Novel Mitovirus of the Sand Fly Lutzomyia Longipalpis Using Genomic and Virus–Host Interaction Signatures
viruses Article Characterization of a Novel Mitovirus of the Sand Fly Lutzomyia longipalpis Using Genomic and Virus–Host Interaction Signatures Paula Fonseca 1 , Flavia Ferreira 2, Felipe da Silva 3, Liliane Santana Oliveira 4,5 , João Trindade Marques 2,3,6 , Aristóteles Goes-Neto 1,3, Eric Aguiar 3,7,*,† and Arthur Gruber 4,5,8,*,† 1 Department of Microbiology, Instituto de Ciências Biológicas, Universidade Federal de Minas Gerais, Belo Horizonte 30270-901, Brazil; [email protected] (P.F.); [email protected] (A.G-N.) 2 Department of Biochemistry and Immunology, Instituto de Ciências Biológicas, Universidade Federal de Minas Gerais, Belo Horizonte 30270-901, Brazil; [email protected] (F.F.); [email protected] (J.T.M.) 3 Bioinformatics Postgraduate Program, Instituto de Ciências Biológicas, Universidade Federal de Minas Gerais, Belo Horizonte 30270-901, Brazil; [email protected] 4 Bioinformatics Postgraduate Program, Universidade de São Paulo, São Paulo 05508-000, Brazil; [email protected] 5 Department of Parasitology, Instituto de Ciências Biomédicas, Universidade de São Paulo, São Paulo 05508-000, Brazil 6 CNRS UPR9022, Inserm U1257, Université de Strasbourg, 67084 Strasbourg, France 7 Department of Biological Science (DCB), Center of Biotechnology and Genetics (CBG), State University of Santa Cruz (UESC), Rodovia Ilhéus-Itabuna km 16, Ilhéus 45652-900, Brazil 8 European Virus Bioinformatics Center, Leutragraben 1, 07743 Jena, Germany * Correspondence: [email protected] (E.A.); [email protected] (A.G.) † Both corresponding authors contributed equally to this work. Citation: Fonseca, P.; Ferreira, F.; da Silva, F.; Oliveira, L.S.; Marques, J.T.; Goes-Neto, A.; Aguiar, E.; Gruber, Abstract: Hematophagous insects act as the major reservoirs of infectious agents due to their intimate A. -
Downloaded a Third Explanation Is That Birds Are Particularly Efficient from Ensembl [34]
Cui et al. Genome Biology 2014, 15:539 http://genomebiology.com/2014/15/12/539 RESEARCH Open Access Low frequency of paleoviral infiltration across the avian phylogeny Jie Cui1,8*†, Wei Zhao2†, Zhiyong Huang2, Erich D Jarvis3, M Thomas P Gilbert4,7, Peter J Walker5, Edward C Holmes1 and Guojie Zhang2,6* Abstract Background: Mammalian genomes commonly harbor endogenous viral elements. Due to a lack of comparable genome-scale sequence data, far less is known about endogenous viral elements in avian species, even though their small genomes may enable important insights into the patterns and processes of endogenous viral element evolution. Results: Through a systematic screening of the genomes of 48 species sampled across the avian phylogeny we reveal that birds harbor a limited number of endogenous viral elements compared to mammals, with only five viral families observed: Retroviridae, Hepadnaviridae, Bornaviridae, Circoviridae, and Parvoviridae. All nonretroviral endogenous viral elements are present at low copy numbers and in few species, with only endogenous hepadnaviruses widely distributed, although these have been purged in some cases. We also provide the first evidence for endogenous bornaviruses and circoviruses in avian genomes, although at very low copy numbers. A comparative analysis of vertebrate genomes revealed a simple linear relationship between endogenous viral element abundance and host genome size, such that the occurrence of endogenous viral elements in bird genomes is 6- to 13-fold less frequent than in mammals. Conclusions: These results reveal that avian genomes harbor relatively small numbers of endogenous viruses, particularly those derived from RNA viruses, and hence are either less susceptible to viral invasions or purge them more effectively. -
Systematic Survey of Non-Retroviral Virus-Like Elements in Eukaryotic
Virus Research 262 (2019) 30–36 Contents lists available at ScienceDirect Virus Research journal homepage: www.elsevier.com/locate/virusres Systematic survey of non-retroviral virus-like elements in eukaryotic genomes T ⁎ Kirill Kryukova, Mahoko Takahashi Uedab, Tadashi Imanishia, So Nakagawaa,b, a Department of Molecular Life Science, Tokai University School of Medicine, 143 Shimokasuya, Isehara, Kanagawa 259-1193, Japan b Micro/Nano Technology Center, Tokai University, 411 Kitakaname, Hiratsuka, Kanagawa 259-1292, Japan ARTICLE INFO ABSTRACT Keywords: Endogenous viral elements (EVEs) are viral sequences that are endogenized in the host cell. Recently, several Endogenous viral elements eukaryotic genomes have been shown to contain EVEs. To improve the understanding of EVEs in eukaryotes, we Database have developed a system for detecting EVE-like sequences in eukaryotes and conducted a large-scale nucleotide Evolution sequence similarity search using all available eukaryotic and viral genome assembly sequences (excluding those Comparative genomics from retroviruses) stored in the National Center for Biotechnology Information genome database (as of August 14, 2017). We found that 3856 of 7007 viral genomes were similar to 4098 of 4102 eukaryotic genomes. For those EVE-like sequences, we constructed a database, Predicted Endogenous Viral Elements (pEVE, http://peve. med.u-tokai.ac.jp) which provides comprehensive search results summarized from an evolutionary viewpoint. A comparison of EVE-like sequences among closely related species may be useful to avoid false-positive hits. We believe that our search system and database will facilitate studies on EVEs. 1. Introduction EVEs in the genomes of various species. However, this rapid increase in genome availability may cause difficulties in the comprehensive detection Virus DNA can become integrated into a host genome, where, if of EVEs in eukaryotic genomes. -
The Discovery, Distribution and Diversity of DNA Viruses Associated with Drosophila Melanogaster in Europe Authors: Megan A
bioRxiv preprint doi: https://doi.org/10.1101/2020.10.16.342956; this version posted October 16, 2020. The copyright holder for this preprint (which was not certified by peer review) is the author/funder, who has granted bioRxiv a license to display the preprint in perpetuity. It is made available under aCC-BY-NC-ND 4.0 International license. DNA viruses of European Drosophila The discovery, distribution and diversity of DNA viruses associated with Drosophila melanogaster in Europe Authors: Megan A. Wallace 1,2 [email protected] 0000-0001-5367-420X Kelsey A. Coffman 3 [email protected] 0000-0002-7609-6286 Clément Gilbert 1,4 [email protected] 0000-0002-2131-7467 Sanjana Ravindran 2 [email protected] 0000-0003-0996-0262 Gregory F. Albery 5 [email protected] 0000-0001-6260-2662 Jessica Abbott 1,6 [email protected] 0000-0002-8743-2089 Eliza Argyridou 1,7 [email protected] 0000-0002-6890-4642 Paola Bellosta 1,8,9 [email protected] 0000-0003-1913-5661 Andrea J. Betancourt 1,10 [email protected] 0000-0001-9351-1413 Hervé Colinet 1,11 [email protected] 0000-0002-8806-3107 Katarina Eric 1,12 [email protected] 0000-0002-3456-2576 Amanda Glaser-Schmitt 1,7 [email protected] 0000-0002-1322-1000 Sonja Grath 1,7 [email protected] 0000-0003-3621-736X Mihailo Jelic 1,13 [email protected] 0000-0002-1637-0933 Maaria Kankare 1,14 [email protected] 0000-0003-1541-9050 Iryna Kozeretska 1,15 [email protected] 0000-0002-6485-1408 Volker Loeschcke 1,16 [email protected] 0000-0003-1450-0754 Catherine Montchamp-Moreau 1,4 [email protected] 0000-0002-5044-9709 Lino Ometto 1,17 [email protected] 0000-0002-2679-625X Banu Sebnem Onder 1,18 [email protected] 0000-0002-3003-248X Dorcas J.