The Not So Identical Twins: (Dis)Similarities Between Reactive Electrophile and Oxidant Sensing and Signaling
Total Page:16
File Type:pdf, Size:1020Kb
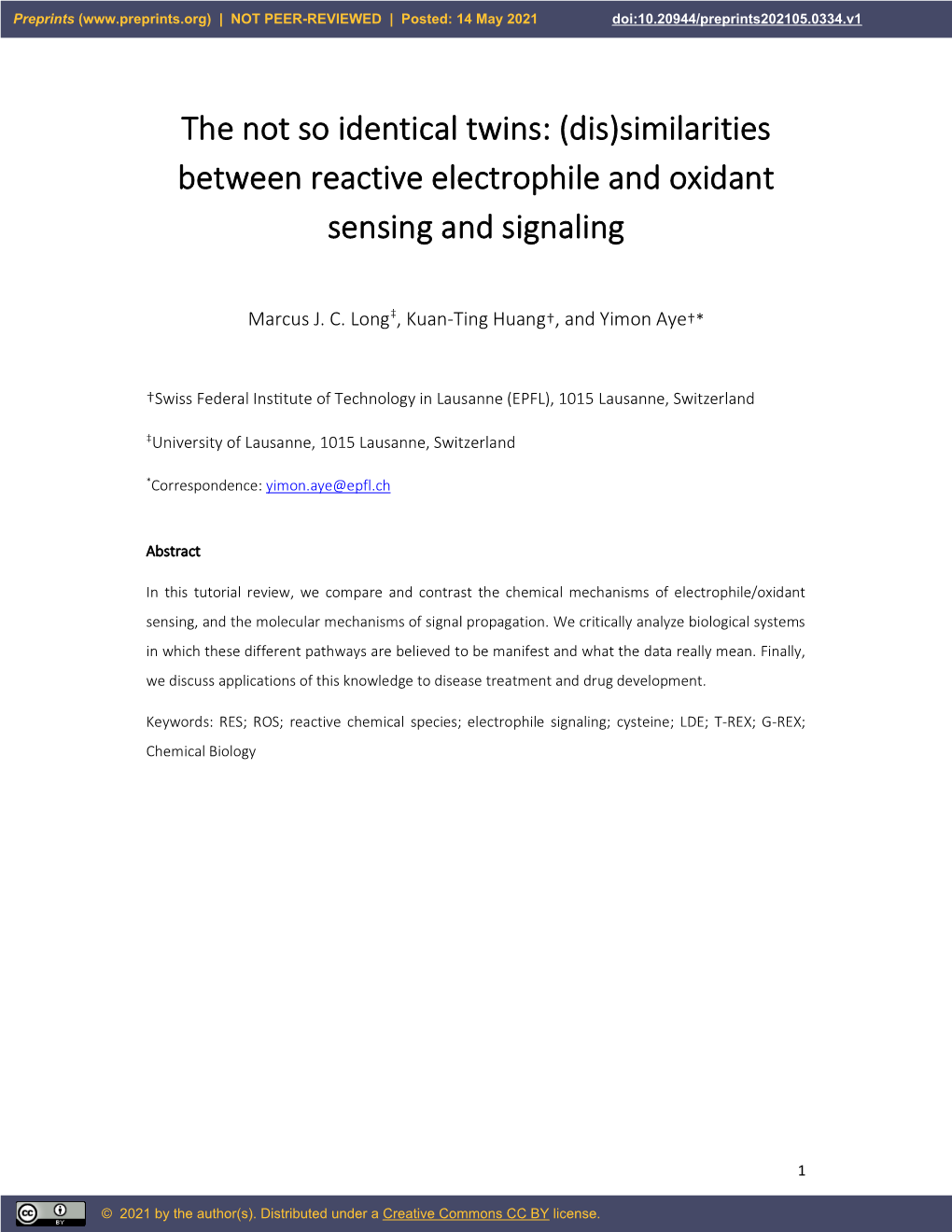
Load more
Recommended publications
-
Genetically Encoded Fragment-Based Discovery
Available online at www.sciencedirect.com ScienceDirect Genetically encoded fragment-based discovery 1 Ratmir Derda and Simon Ng This opinion describes recent advances of molecular discovery the fragment of interest. This opinion focuses on chemi- technology dubbed Genetically Encoded Fragment-Based cal post-translational incorporation of fragments Discovery (GE-FBD). GE-FBD starts from a known ligand or (Figure 1b). Alternatively, {FP} libraries can be built from ‘fragment’ that binds to a desired target weakly and often with unnatural amino acids (UAA) that contain fragments as low specificity. Covalent incorporation of fragment into a part of their side-chain (Figure 1c). Selection from {FP} diverse, genetically encoded library of peptides yields a library libraries has been shown to identify ligands in which the of peptide–fragment combinations. Selection from such a fragment covalently linked to peptide segment bind the library has a high likelihood to identify ligands, in which the target with improved affinity and specificity when com- peptides bind to distinct adjacent pockets of the target in pared to the original fragment (Figure 1d). Improvement F FP synergy with the fragment and exhibits enhanced affinity and can be defined as r = KD/ KD > 1 or DDGbind Àlog F specificity when compared to the fragment itself. GE-FBD (r) < 0, where KD is the affinity of the fragment towards could employ fragments that bind non-covalently as well as the target of interest (Figure 1e). Figure 1d summarizes reversible covalent warheads. The key advances in GE-FBD up-to-date outcomes from published GE-FBD reports include (i) synthetic chemistry that enables incorporation of and shows that r = 10–100 can be found across diverse F diverse fragments into both linear and cyclic peptide libraries; fragment with KD ranging from 100 nM to 1 mM [3 ,4– (ii) quantification of multi-step modifications in million-to-billion 6,7 ,8,9 ,10,11 ,12,13 ,14]. -
ICBS 2018 Vancouver, Canada
7th Annual Conference September 24-27, 2018 ICBS 2018 Vancouver, Canada Scientific Program Towards Translational Impact www.chemical-biology.org 7th Annual Conference | September 24-27, 2018 | Vancouver, Canada Acknowledgements Towards Translational Impact September 24th – 27th, 2018 | Vancouver, BC Local Program and Organizing Committee Tom Pfeifer, Centre for Drug Research and Development Roger Linington, Simon Fraser University Michel Roberge, University of British Columbia Nicolette Honson, Centre for Drug Research and Development ICBS Organizing Committee Haian Fu, Chair, Emory University, USA Lixin Zhang, ECUST, China Sally-Ann Poulsen, Griffith University, Australia Jonathan Baell, Monash University, Australia Mahabir Gupta, University of Panama, Panama Junying Yuan, Harvard Medical School, USA Masatoshi Hagiwara, Kyoto University, Japan Petr Bartunek, CZ-OPENSCREEN and Institute of Molecular Jason Micklefield, The University of Manchester, UK Genetics, Czech Republic Siddhartha Roy, Boss Institute, India ICBS Young Chemical Biologist Award 2018 Selection Committee Yimon Aye, Cornell University, USA Christian Ottmann, Technische Universiteit Eindhoven, Ratmir Derda, University of Alberta, Canada Netherlands Evripidis Gavathiotis, Albert Einstein College of Medicine, USA William Pomerantz, University of Minnesota, USA Kenjiro Hanaoka, University of Tokyo, Japan Qi Zhang, Fudan University, China Recording of sessions (oral or poster) by audio, video, or still photography is strictly prohibited except with the advance permission of -
California Insɵtute of Technology Division of Biology and Biological Engineering Annual Report 2017
California InsƟtute of Technology Division of Biology and Biological Engineering Annual Report 2017 Introduction Annual Report | Biology and Biological Engineering | 2017 Introduction The annual report for Caltech's Division of Biology and Biological Engineering (BBE) presents major research accomplishments of faculty, students, and staff during the previous academic year. This report covers October 1, 2016 to September 30, 2017. Front Cover Illustration Vector-assisted spectral tracing (VAST) in the cerebellum of an adult mouse A movie highlighting the multi-color vector-assisted spectral tracing (VAST) system in the cerebellum of an adult mouse. Due to the stochastic uptake of AAV-PHP viruses encoding either a blue, green or red fluorescent protein, cells are labeled with a wide range of hues. This approach can be used to differentiate neighboring neurons for morphology and tracing studies. Credit: Ben Deverman et al., Gradinaru Lab Back Cover Illustration Engineered adeno-associated viruses efficiently cross the blood-brain-barrier for enhanced brain transduction in adult mice. Representative images of virally-delivered nuclear GFP fluorescence (green) and Calbindin immunohistochemistry (magenta) in the cerebellum. Credit: Chan et al., Gradinaru Lab 1 News, Events, and People Annual Report | Biology and Biological Engineering | 2017 Press Releases 5 Annual Retreat 14 Ferguson Prize 16 Ferguson Prize 17 Professorial Awards and Honors 18 Seminars 19 Named Lectures 25 2 News, Events, and People Annual Report | Biology and Biological Engineering -
A Tipping Point in Cancer Research
A Tipping Point in Cancer Research Annual Report 2011 IN THIS NEW ERA OF ACCELERATED PROGRESS, THEIR INNOVATIVE CONTRIBUTIONS HAVE it is more important than ever that we support BEEN WIDELY RECOGNIZED: creative young scientists who can harness the • 2 Damon Runyon scientists were included in A Tipping Point in Cancer Research momentum of the past decade to transform the Science magazine’s Insights of the Decade, way we prevent, detect, and treat cancer. The Celebrating Extraordinary Progress which listed “10 insights that have changed science Damon Runyon Cancer Research Foundation is since the dawn of the new millennium.” SCIENTISTS, RESEARCH ORGANIZATIONS AND THE NATIONAL CANCER INSTITUTE ALL AGREE: playing a central role by funding groundbreaking we are at a tipping point in cancer research. The convergence of knowledge from across the spectrum basic and clinical cancer research. In the past year, • 2 Damon Runyon alumni were elected to the of cutting-edge science combined with powerful new technologies has created a deeper understanding our brilliant young scientists: National Academy of Sciences (the science “Hall of Fame”), bringing Damon Runyon’s total to 61. of cancers and advanced our ability to detect and treat these diseases earlier and more effectively. • led studies resulting in FDA approval of two new drugs for advanced melanoma: Yervoy, an immu- • William R. Sellers, MD, Board Member and notherapy, and Zelboraf, a targeted therapy former Clinical Investigator, was appointed by President Obama to the National Cancer • reported the success of a novel immunotherapy Advisory Board. for pancreatic cancer • Elaine V. Fuchs, PhD, Board Member and • completed the first genome sequencing of former Fellow, received the prestigious Albany multiple myeloma and medulloblastoma Medical Center Prize. -
Current Research in Chemical Biology
CURRENT RESEARCH IN CHEMICAL BIOLOGY AUTHOR INFORMATION PACK TABLE OF CONTENTS XXX . • Description p.1 • Editorial Board p.2 • Guide for Authors p.3 ISSN: 2666-2469 DESCRIPTION . Current Research in Chemical Biology (CRCHBI) is a new primary research, gold open access journal from Elsevier. CRCHBI publishes original papers and short communications that cover all aspects of chemical biology and related areas. Current Research in Chemical Biology is a peer-reviewed gold open access (OA) journal and upon acceptance all articles are permanently and freely available. It is a companion to the highly regarded review journal Current Opinion in Chemical Biology and is part of the Current Opinion and Research (CO+RE) suite of journals. All CO+RE journals leverage the Current Opinion legacy- of editorial excellence, high-impact, and global reach-to ensure they are a widely read resource that is integral to scientists' workflow. Current Research in Chemical Biology topics covered include: Bioorganic and bioinorganic chemistry Biocatalysis and biotransformation (Applied) medicinal chemistry Next generation therapeutics (e.g. PROTACs, protein/peptide-based therapeutics, ..) Molecular imaging, high resolution methods in microscopy Synthetic biology and biomolecules Energy (e.g. biological pathways for electrons, protons and photo-excitations) Mechanistic biology Genome- and proteome-wide analysis Metabolomics Assay development Photo-induced processes and light switches Current Research in Chemical Biologybuilds on Elsevier's reputation for excellence in scientific publishing. Expertise - Editors and Editorial Board bring depth and breadth of expertise and experience to the journal. Speed - Submission and peer review is fast, and publication of final manuscripts is instantaneous. Discoverability - Articles get high visibility and maximum exposure on an industry-leading platform that reaches a vast global audience. -
COURSE SCHEDULE: Biochemistry/Chemistry 945: Seminar—Chemical Biology (Advanced) Blackwell — Spring 2018 Wednesdays @ 4:35 Pm, Room 8335 Chemistry
COURSE SCHEDULE: Biochemistry/Chemistry 945: Seminar—Chemical Biology (Advanced) Blackwell — Spring 2018 Wednesdays @ 4:35 pm, Room 8335 Chemistry Discussion Date Speaker Paper CBI-relevant Seminars Facilitator Jan Introduction Blackwell None Paula Hammond – Jan 29/30 24 Gut microbiome modulates response to anti-PD-1 immunotherapy in melanoma Jan Marc Currie patients; Wargo, Science, 2018, 359, 97–103; Herman Sintim - Jan 26 31 Chevrette Commentary: Jobin Science, 2018, 359, 32–34 Polyamine-Mediated Stoichiometric Assembly of Ribonucleoproteins for Benjamin Feb 7 Lynn Enhanced mRNA Delivery Ortiz Hammond, Angew Chem Int Ed, 2017, 56, 13709–13712 Acoustic reporter genes for noninvasive imaging of microorganisms in Feb Emily Cai mammalian hosts 14 Ehlerding Shapiro, Nature, 2018, 553, 86–90 Akt3 is a privileged first responder in isozyme-specific electrophile response Feb Mark Klein Denu Aye, Nature Chem Bio, 2017, 13, 333–338; 21 Commentary: Weerapana, Nature Chem Bio, 2017, 13, 244–245 Programmable base editing of A•T to G•C in genomic DNA without DNA Feb Rebeca Brunold cleavage Yimon Aye - Feb 27 28 Fernandez Liu, Nature, 2017, 551, 464–471 Tim Multidimensional chemical control of CRISPR-Cas9 Mar 7 Jin Tiambeng Shoulders & Choudhary, Nature Chem Bio, 2017, 13, 9–11 An Approach to Spatiotemporally Resolve Protein Interaction Networks in Living Mar Trisha Ge Cells Matt Shoulders – Mar 13 14 Tucholski Krogan, Cell, 2017, 169, 350–360 A semi-synthetic organism that stores and retrieves increased genetic Mar Joseph Blackwell information 21 -
3.3-Å Resolution Cryo-EM Structure of Human Ribonucleotide Reductase with Substrate and Allosteric Regulators Bound
3.3-Å resolution cryo-EM structure of human ribonucleotide reductase with substrate and allosteric regulators bound The MIT Faculty has made this article openly available. Please share how this access benefits you. Your story matters. Citation Brignole, Edward J et al. “3.3-Å Resolution Cryo-EM Structure of Human Ribonucleotide Reductase with Substrate and Allosteric Regulators Bound.” eLife 7 (February 2018): e31502 © Brignole et al As Published http://dx.doi.org/10.7554/eLife.31502 Publisher eLife Sciences Publications, Ltd Version Final published version Citable link http://hdl.handle.net/1721.1/114937 Terms of Use Creative Commons Attribution 4.0 International License Detailed Terms https://creativecommons.org/licenses/by/4.0/ RESEARCH ARTICLE 3.3-A˚ resolution cryo-EM structure of human ribonucleotide reductase with substrate and allosteric regulators bound Edward J Brignole1,2†, Kuang-Lei Tsai3†‡, Johnathan Chittuluru3, Haoran Li4, Yimon Aye4§, Pawel A Penczek5, JoAnne Stubbe2,4*, Catherine L Drennan1,2,4*, Francisco Asturias3#* 1Howard Hughes Medical Institute, Massachusetts Institute of Technology, Cambridge, United States; 2Department of Biology, Massachusetts Institute of Technology, Cambridge, United States; 3Department of Integrative Computational and Structural Biology, The Scripps Research Institute, La Jolla, United States; *For correspondence: 4Department of Chemistry, Massachusetts Institute of Technology, Cambridge, [email protected] (JAS); 5 [email protected] (CLD); United States; Department of Biochemistry and Molecular Biology, The University [email protected] of Texas-Houston Medical School, Houston, United States (FA) †These authors contributed equally to this work Abstract Ribonucleotide reductases (RNRs) convert ribonucleotides into deoxyribonucleotides, Present address: ‡Department a reaction essential for DNA replication and repair. -
Table of Contents (PDF)
June 14, 2011 u vol. 108 u no. 24 u 9727–10022 Cover image: Pictured is the ladybird beetle Delphastus catalinae next to the rim of a US one cent coin. Stephen T. Deyrup and colleagues used 2D NMR spectroscopy to screen metabolites, including defensive polyketides that these tiny beetles produce. The technique revealed partial molecular structures that allowed the authors to identify novel compounds within the complex mixtures without prior fractionation or isolation. See the article by Deyrup et al. on pages 9753–9758. Image courtesy of Frank C. Schroeder. From the Cover 9753 Searching for new chemical entities 9776 Clay minerals and carbon sequestration 9782 Innate grasp of Euclidean geometry 9851 Targeting cancer with hyperthermia 9910 Speciation among whiptail lizards 9733 Spontaneous speciation by ploidy elevation: Laboratory Contents synthesis of a new clonal vertebrate Craig Moritz and Ke Bi See companion article on page 9910 THIS WEEK IN PNAS 9727 In This Issue PNAS PLUS (AUTHOR SUMMARIES) LETTERS (ONLINE ONLY) BIOLOGICAL SCIENCES E198 Algal ice-binding proteins change the structure of sea ice CELL BIOLOGY James A. Raymond 9735 Targeting and imaging single biomolecules in living cells E199 ADAR1 isoform involvement in embryonic lethality by complementation-activated light microscopy with Richard A. Steinman and Qingde Wang split-fluorescent proteins Fabien Pinaud and Maxime Dahan E200 Reply to Steinman and Wang: Involvement of the p150 See full research article on page E201 of www.pnas.org isoform of the RNA editing enzyme ADAR1 in embryonic lethality Simone V. Ward, Cyril X. George, Charles E. Samuel, PHYSIOLOGY and Michael B. -
Where Electrophile Signaling and Covalent Ligandâœtarget Mining
News from New Chemistry Professors iN switzerlaNd CHIMIA 2020, 74, No. 9 659 doi:10.2533/chimia.2020.659 Chimia 74 (2020) 659–666 © Y. Aye Where Electrophile Signaling and Covalent Ligand–Target Mining Converge Yimon Aye* Abstract: Interests in learning how to engineer most effective covalent ligands, identify novel functional targets, and define precise mechanism-of-action are rapidly growing in both academia and pharmaceutical industries. We here illuminate the establishment of a multifunctional platform that offers new capabilities to logically engineer covalent ligands and dissect ‘on-target’ bioactivity with precise biological context and precision hitherto inac- cessible. Broadly aimed at non-specialist readers, this opinion piece is aimed to stoke the interest of emerging chemists and biologists/bioengineers, but the underlying technological and conceptual topicality is anticipated to also appeal to experts leading ligand–target mining, validation, and -discovery research programs. Keywords: Covalent ligands · Electrophile signalling · Ligand–Target mining Yimon Aye read chemistry at University trophilicity with respect to Group 1. Tecfidera, Vumerity,[6] and of Oxford (UK) (2000–04), and received plant-derived compounds such as curcumin and sulphoraphane her PhD degree in organic chemistry with belong to Group 2 (Fig. 1a). Generally, from reactivity–selectivity David Evans (Harvard, USA) and postdoc- principles, Group 2 compounds of magnified electrophilicity are toral training in biochemistry with JoAnne anticipated to cover a significantly broader on-/off-target spectra. Stubbe (MIT, USA), where she established the mode-of-action of therapeutics targeting When Natural and Unnatural Worlds Converge ribonucleotide reductase. In her indepen- Interestingly, Nature has endowed us with a repertoire of elec- dent career that began mid-2012, she set trophilic small-molecule metabolites having similar properties out to understand the detailed mechanisms to Group 2 molecules. -
Chemical Biology Volume 48 (2019 ) Editor Benjamin G Davis UK
Current Opinion in Chemical Biology Volume 48 ( 2019 ) Editor Benjamin G Davis UK Editorial Enquiries Editorial Board 2019 Contents Current Opinion in Chemical Biology Duilio Arigoni (Switzerland) The subject of chemical biology is divided into Elsevier Jacqueline K. Barton (USA) nine major sections, each of which is reviewed once a year. Each issue contains one or two of the Radarweg 29 Hagan Bayley (UK) sections, and the amount of space devoted to Stephen J. Benkovic (USA) 1043 NX Amsterdam each section is related to its importance. The Netherlands Carolyn Bertozzi (USA) Tel.: +31 (0)20 485 2560 Thomas Cech (USA) e-mail: [email protected] Michelle Chang (USA) February Peng Chen (China) Jason Chin (UK) Omics Benjamin F. Cravatt (USA) Edited by Ileana M. Cristea and Kathryn S. Lilley Current Opinion in Chemical Biology William F. DeGrado (USA) ISSN 1367-5931 Peter B. Dervan (USA) is published bimonthly by Alan R. Fersht (UK) April Elsevier Katharina Gaus (Australia) www.elsevier.com Biocatalysis and Biotransformation Harry B. Gray (USA) Edited by Kylie Vincent and Bettina Nestl Jay Groves (USA) The Aims of the journal can be found at Bioinorganic Chemistry Itaru Hamachi (Japan) Edited by Kyle Lancaster www.elsevier.com/locate/cbi Chuan He (USA) Paul J. Hergenrother (USA) Current Opinion in Chemical Biology Donald Hilvert (Switzerland) June is indexed and/or abstracted by Philipp Holliger (UK) BIOSIS Linda Hsieh-Wilson (USA) Next Generation Therapeutics Edited by Paul Hergenrother and Yimon Aye CAB Abstracts International Barbara Imperiali (USA) CAB Health J. Bryan Jones (Canada) Daniel Kahne (USA) Chemical Abstracts August EMBASE Stephen H.B. -
Clofarabine Targets the Large Subunit (A) of Human Ribonucleotide Reductase in Live Cells by Assembly Into Persistent Hexamers
View metadata, citation and similar papers at core.ac.uk brought to you by CORE provided by Elsevier - Publisher Connector Chemistry & Biology Brief Communication Clofarabine Targets the Large Subunit (a) of Human Ribonucleotide Reductase in Live Cells by Assembly into Persistent Hexamers Yimon Aye,1 Edward J. Brignole,1,3,4 Marcus J.C. Long,5 Johnathan Chittuluru,4 Catherine L. Drennan,1,2,3 Francisco J. Asturias,4 and JoAnne Stubbe1,2,* 1Department of Chemistry 2Department of Biology 3Howard Hughes Medical Institute Massachusetts Institute of Technology, Cambridge, MA 02139, USA 4Department of Cell Biology, The Scripps Research Institute, La Jolla, CA 92037, USA 5Graduate Program in Biochemistry and Biophysics, Brandeis University, Waltham, MA 02454, USA *Correspondence: [email protected] http://dx.doi.org/10.1016/j.chembiol.2012.05.015 SUMMARY yet unresolved, active complex(es) proposed to be a2b2, a6b2, and/or a6b6 (Kashlan and Cooperman, 2003; Rofougaran et al., Clofarabine (ClF) is a drug used in the treatment 2006; Fairman et al., 2011; Hofer et al., 2012). In addition to of leukemia. One of its primary targets is human the NDP-binding, catalytic (C) site, (a2)m possesses two well- ribonucleotide reductase (hRNR), a dual-subunit, characterized allosteric sites. The activity (A) site, within the N-terminal ATP-cone-domain, binds either ATP, activating (a2)m(b2)n, regulatory enzyme indispensable in de novo dNTP synthesis. We report that, in live mam- RNR activity, or dATP, inhibiting it. The specificity (S) site, at a malian cells, ClF targets hRNR by converting its the 2 interface, binds dNTP/ATP, modulating the substrate preference at the C site (Fairman et al., 2011). -
3.3-A˚Resolution Cryo-EM Structure of Human Ribonucleotide Reductase
RESEARCH ARTICLE 3.3-A˚ resolution cryo-EM structure of human ribonucleotide reductase with substrate and allosteric regulators bound Edward J Brignole1,2†, Kuang-Lei Tsai3†‡, Johnathan Chittuluru3, Haoran Li4, Yimon Aye4§, Pawel A Penczek5, JoAnne Stubbe2,4*, Catherine L Drennan1,2,4*, Francisco Asturias3#* 1Howard Hughes Medical Institute, Massachusetts Institute of Technology, Cambridge, United States; 2Department of Biology, Massachusetts Institute of Technology, Cambridge, United States; 3Department of Integrative Computational and Structural Biology, The Scripps Research Institute, La Jolla, United States; *For correspondence: 4Department of Chemistry, Massachusetts Institute of Technology, Cambridge, [email protected] (JAS); 5 [email protected] (CLD); United States; Department of Biochemistry and Molecular Biology, The University [email protected] of Texas-Houston Medical School, Houston, United States (FA) †These authors contributed equally to this work Abstract Ribonucleotide reductases (RNRs) convert ribonucleotides into deoxyribonucleotides, Present address: ‡Department a reaction essential for DNA replication and repair. Human RNR requires two subunits for activity, of Biochemistry and Molecular the a subunit contains the active site, and the b subunit houses the radical cofactor. Here, we Biology, The University of Texas- present a 3.3-A˚ resolution structure by cryo-electron microscopy (EM) of a dATP-inhibited state of Houston Medical School, human RNR. This structure, which was determined in the presence of substrate CDP and allosteric Houston, United States; regulators ATP and dATP, has three a units arranged in an a ring. At near-atomic resolution, §Department of Chemistry & 2 6 these data provide insight into the molecular basis for CDP recognition by allosteric specificity Chemical Biology, Cornell University, Ithaca, United States; effectors dATP/ATP.