Influence of Native Freshwater Mussel Functional Traits And
Total Page:16
File Type:pdf, Size:1020Kb
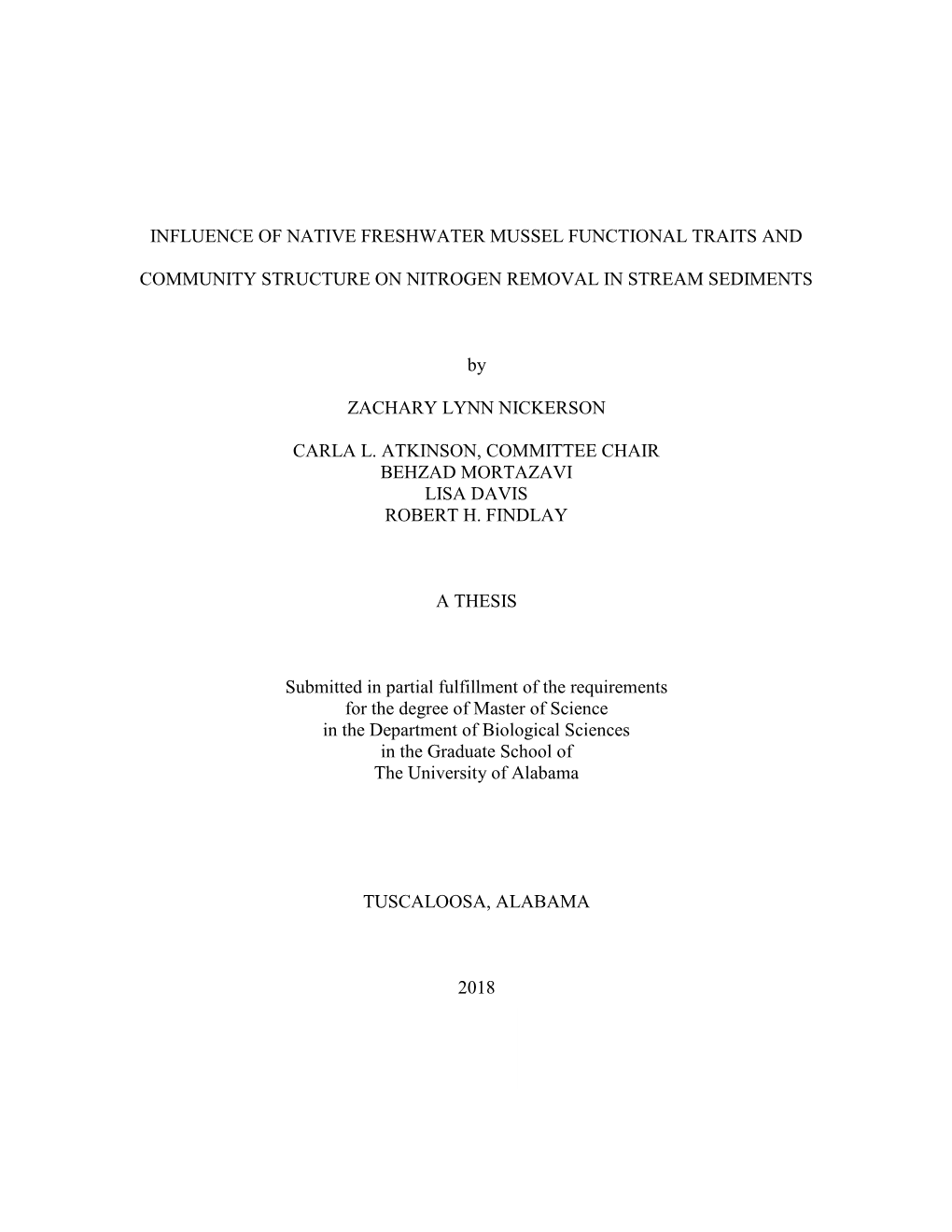
Load more
Recommended publications
-
Caracara Cheriway
Rare Animal Fact Sheet IMBIV31060 Louisiana Department of Wildlife and Fisheries Natural Heritage Program Obovaria unicolor Alabama Hickorynut Identification: This mussel has a round or elliptical shape; shell is nearly smooth, shiny, brown to yellowish brown with rays, young shells are green with green rays; mother-of-pear is pink but sometimes white or blue. Measurements: A small mussel, 1.5 to 2 inches in length. Taxonomic comments: No recognized subspecies. Status: Global ranking is G3 and state rank is S1. Habitat: Sand and gravel bottoms in river systems. Range: Eastern gulf drainages throughout Alabama, Louisiana, Mississippi, and Oklahoma. Food habits*: Mussels are continually pumping water through their siphon. Their diet is composed of the various micropscopic plants and animals from the water and organic matter from stream bottoms that they filter from this water. Reproduction: Sexes are separate. No information on host fish available. Reason for decline: 1) The damming of stream and rivers results in the loss of mussel and host fish habitat. 2) Channelization of large stream habitat. 3) Declining water quality as a result of siltation (reduces oxygen level) and heavy recreational use of habitat. Interesting facts: This species is common only in the Sipsey River in Alabama. It is believed to have been uncommon or historically rare throughout the rest of its range. * Indicates generalized information for freshwater mussels in the absence of information specific to this species Rare Animal Fact Sheet IMBIV31060 Known distribution in Louisiana: Dates of documented observations are: 1977, 1979, 1988, and 1994 References McCullagh, W.H., J.D. Williams, S.W. -
The Gut Microbiome of Freshwater Unionidae Mussels Is Determined by Host Species and Is Selectively Retained from Filtered Seston
University of Mississippi eGrove Faculty and Student Publications Biology 11-1-2019 The gut microbiome of freshwater Unionidae mussels is determined by host species and is selectively retained from filtered seston Eric A. Weingarten University of Mississippi Carla L. Atkinson University of Alabama Colin R. Jackson University of Mississippi Follow this and additional works at: https://egrove.olemiss.edu/biology_facpubs Recommended Citation Weingarten, E. A., Atkinson, C. L., & Jackson, C. R. (2019). The gut microbiome of freshwater Unionidae mussels is determined by host species and is selectively retained from filtered seston. PLOS ONE, 14(11), e0224796. https://doi.org/10.1371/journal.pone.0224796 This Article is brought to you for free and open access by the Biology at eGrove. It has been accepted for inclusion in Faculty and Student Publications by an authorized administrator of eGrove. For more information, please contact [email protected]. RESEARCH ARTICLE The gut microbiome of freshwater Unionidae mussels is determined by host species and is selectively retained from filtered seston 1 2 1 Eric A. Weingarten , Carla L. Atkinson , Colin R. JacksonID * 1 Department of Biology, University of Mississippi, University, Mississippi, United States of America, 2 Department of Biological Sciences, University of Alabama, Tuscaloosa, Alabama, United States of America * [email protected] a1111111111 a1111111111 a1111111111 Abstract a1111111111 a1111111111 Freshwater mussels are a species-rich group of aquatic invertebrates that are among the most endangered groups of fauna worldwide. As filter-feeders that are constantly exposed to new microbial inoculants, mussels represent an ideal system to investigate the effects of species or the environment on gut microbiome composition. -
Download BALMNH No 25 2007
••• 11111111 ••• ••• ... .... ... ••• ALABAMA MUSEUM of Natural History Bulletin 25 August 1, 2007 Systematics, Evolution and Biogeography of the Etheostoma simoterum Species Complex (Percidae: Subgenus Ulocentra) Distribution and Satus of Freshwater Mussels (Bivalvia Unionidae) of the Lower Coosa and Tallapoosa River Drainages in Alabama The Osteology of the Stonecat, Noturus flavus (Siluriformes: Ictaluridae), with Comparisons to Other Siluriforms 8 1 BULLETIN ALABAMA MUSEUM OF NATURAL mSTORY The scientific publication of the Alabama Museum of Natural History. Dr. Phillip Harris, Editor. BULLETIN AlABAMA MUSEUM OF NATURAL HISTORY is published by the Alabama Museum of Natural History, a unit of The University of Alabama. The BULLETIN succeeds its predecessor, the MUSEUM PAPERS, which was terminated in 1961 upon the transfer of the Museum to the University from its parent organiza tion, the Geological Survey of Alabama. The BULLETIN is devoted primarily to scholarship and research concerning the natural history of Alabama and the Southeast. It appears twice yearly in consecutively numbered issues. Communication concerning manuscripts, style, and editorial policy should be addressed to: Editor, BULLETIN AlABAMA MUSEUM OF NATURAL HISTORY, The University of Alabama, Box 870345, Tuscaloosa, Alabama 35487-0345; telephone (205) 348-1831 or [email protected]. Prospective authors should exam ine the Notice to Authors inside the back cover. Orders and requests for general information should be addressed to BULLETIN AlABAMA MUSEUM OF NATURAL HISTORY, at the above address or emailed to [email protected]. Yearly subscriptions (two issues) are $30.00 for individ uals, $50.00 for corporations and institutions. Numbers may be purchased individual ly. Payment should accompany orders and subscriptions and checks should be made out to "The University of Alabama." Library exchanges should be handled through: Exchange Librarian, The University of Alabama, Box 870266, Tuscaloosa, Alabama 35487-0340. -
A Review of the Interactions Between Catfishes and Freshwater Mollusks
American Fisheries Society Symposium 77:733–743, 2011 © 2011 by the American Fisheries Society A Review of the Interactions between Catfi shes and Freshwater Mollusks in North America JEREMY S. TIEMANN* Illinois Natural History Survey Institute of Natural Resource Sustainability at the University of Illinois Urbana-Champaign 1816 South Oak Street, Champaign, Illinois 61820, USA STEPHEN E. MCMURRAY Missouri Department of Conservation, Resource Science Division 1110 South College Avenue, Columbia, Missouri 65201, USA M. CHRISTOPHER BARNHART Missouri State University, Department of Biology 901 South National, Springfi eld, Missouri 65897, USA G. THOMAS WATTERS The Ohio State University, Department of Evolution, Ecology, and Organismal Biology 1315 Kinnear Road, Columbus, Ohio 43212, USA Abstract.—Catfi shes are important in freshwater ecosystems not only as consumers, but also as essential partners in symbiotic relationships with other organisms. Freshwater mol- lusks are among the many organisms that have interactions with catfi shes. For example, icta- lurids are hosts for larvae of several native freshwater mussel species. The larvae, which attach briefl y to gills or fi ns of fi sh to complete their development to the free-living juvenile stage, disperse via upstream and downstream movement of host fi sh. In turn, freshwater mussels serve as a food source for some catfi sh species while other catfi sh species may use spent mus- sel shells for habitat. Ictalurids also benefi t from the conservation status of many freshwater mussel species. Federal and state laws protecting these invertebrates can preserve water qual- ity and habitat and, at times, provide incentives and funding for conservation and restoration of stream and riparian habitats. -
Detroit Edison Company One Energy Plaza, Detroit, MI 48226-1279
The Detroit Edison Company One Energy Plaza, Detroit, MI 48226-1279 10 CFR 51.45 10 CFR 52.77 January 29, 2010 NRC3-10-0004 U. S. Nuclear Regulatory Commission Attention: Document Control Desk Washington, DC 20555-0001 References: 1) Fermi 3 Docket No.: 52-033 2) Letter from Bruce Olson (USNRC) to Peter W. Smith (Detroit Edison), "Requests for Additional Information Letter No. 2 Related to the Environmental Review for the Combined License Application for Fermi Nuclear Power Plant, Unit 3," dated November 6, 2009 3) Letter from Peter W. Smith (Detroit Edison) to USNRC, "Detroit Edison Company Response to NRC Requests for Additional Information Related to the Environmental Review," NRC3-09-0014 diated September 30, 2009 4) Letter from Peter W. Smith (Detroit Edison) to USNRC, "Detroit Edison Company Response to NRC Requests for Additional Information Related to the Environmental Review," NRC3-09-0015 dated October 30, 2009 5) Letter from Peter W. Smith (Detroit Edison) to USNRC, "Detroit Edison Company Response to NRC Requests for Additional Information Related to the Environmental Review," NRC3-09-0016 dated November 23, 2009 6) Letter from Peter W. Smith (Detroit Edison) to USNRC, "Detroit Edison Company Response to NRC Requests for Additional Information Related to the Environmental Review," NRC3-09-0017 dated December 23, 2009 Subject: Detroit Edison Company Response to NRC Requests for Additional Information Letter No. 2 Related to the Environmental Review -T)C)9!S 0 go A DTE Energy Company USNRC NRC3-10-0004 Page 2 In Reference 2, the NRC requested additional information to support the review of Part 3 (Environmental Report) of the Fermi 3 Combined License Application (COLA). -
Rare Animals Tracking List
Louisiana's Animal Species of Greatest Conservation Need (SGCN) ‐ Rare, Threatened, and Endangered Animals ‐ 2020 MOLLUSKS Common Name Scientific Name G‐Rank S‐Rank Federal Status State Status Mucket Actinonaias ligamentina G5 S1 Rayed Creekshell Anodontoides radiatus G3 S2 Western Fanshell Cyprogenia aberti G2G3Q SH Butterfly Ellipsaria lineolata G4G5 S1 Elephant‐ear Elliptio crassidens G5 S3 Spike Elliptio dilatata G5 S2S3 Texas Pigtoe Fusconaia askewi G2G3 S3 Ebonyshell Fusconaia ebena G4G5 S3 Round Pearlshell Glebula rotundata G4G5 S4 Pink Mucket Lampsilis abrupta G2 S1 Endangered Endangered Plain Pocketbook Lampsilis cardium G5 S1 Southern Pocketbook Lampsilis ornata G5 S3 Sandbank Pocketbook Lampsilis satura G2 S2 Fatmucket Lampsilis siliquoidea G5 S2 White Heelsplitter Lasmigona complanata G5 S1 Black Sandshell Ligumia recta G4G5 S1 Louisiana Pearlshell Margaritifera hembeli G1 S1 Threatened Threatened Southern Hickorynut Obovaria jacksoniana G2 S1S2 Hickorynut Obovaria olivaria G4 S1 Alabama Hickorynut Obovaria unicolor G3 S1 Mississippi Pigtoe Pleurobema beadleianum G3 S2 Louisiana Pigtoe Pleurobema riddellii G1G2 S1S2 Pyramid Pigtoe Pleurobema rubrum G2G3 S2 Texas Heelsplitter Potamilus amphichaenus G1G2 SH Fat Pocketbook Potamilus capax G2 S1 Endangered Endangered Inflated Heelsplitter Potamilus inflatus G1G2Q S1 Threatened Threatened Ouachita Kidneyshell Ptychobranchus occidentalis G3G4 S1 Rabbitsfoot Quadrula cylindrica G3G4 S1 Threatened Threatened Monkeyface Quadrula metanevra G4 S1 Southern Creekmussel Strophitus subvexus -
In Mississippi National Forests
SUMMARY Little is known about the distribution of freshwater mussels in Mississippi national forests. Review of the scant available infor- mation revealed that the national forests harbor a diverse mus- sel fauna of possibly 46 or more species (including confirmed, probable, and potential occurrences). Occurrence of 33 species is confirmed. Because of the geographic, physiographic, and drain- age basin diversity of Mississippi national forests, there is con- siderable variation in mussel communities among the national forests. Three distinct fauna1 groups are represented in Missis- sippi national forests, each with a characteristic assemblage of species. One species of potential occurrence is a federally endan- gered species, 1 species of confirmed occurrence is a candidate for listing, and 11 species of confirmed or probable occurrence are considered of special concern by the American Fisheries Society (Williams and others 1993). None of the national forests have been surveyed adequately, and specific population data are almost com- pletely lacking. This review of existing information represents the first of a three-phase program needed to comprehensively evalu- ate the mussel resources of Mississippi national forests. Phase two involves an exhaustive, qualitative field survey of Mississippi national forests to document precise distribution of species and location of important communities. Phase three consists of a quan- titative study of important communities in order to assess repro- ductive characteristics and viability and to establish baseline density estimates for monitoring of future population trends. Cover: left, Lampsilis cardium; top right, Utterbackia imbecillis; bottom right, Potamilus ohiensis. Current Distributional Information on Freshwater Mussels (family Unionidae) in Mississippi National Forests Wendell R. -
Species Status Assessment Report for the Round Hickorynut Mussel (Obovaria Subrotunda)
Species Status Assessment Report for the Round Hickorynut Mussel (Obovaria subrotunda) Photo credit: Environment Canada October 2019 Version 1.0 U.S. Fish and Wildlife Service Region 4 Atlanta, GA 1 Primary Contributors • Andrew Henderson - Asheville Field Office, Lead Biologist (Region 4) – primary author • Heidi Crowell - Pacific Southwest Regional Office, SAT Project Manager (Region 8) • Mark Endries - Asheville Field Office (Region 4) – mapping support Contributors & Agency Reviewers (underlined) • Paul Hartfield, Leroy Koch (retired), Angela Boyer, Stephanie Chance (retired), Bob Anderson, Tyler Hern, Andy Ford, Patty Morrison (retired), Bob Butler (retired), Josh Hundley (U.S. Fish and Wildlife Service) • Dr. Todd Morris (Fisheries & Oceans Canada) • Chuck Howard (retired), Tim Keeling (Tennessee Valley Authority) • Kierstin Carlson (Western Pennsylvania Conservancy) • Steve Ahlstedt (retired) (U.S. Geological Survey) • Dr. Arthur Bogan, Jamie Smith (North Carolina Museum of Natural Sciences) • Jeremy Tiemann, Rachel Vinsel, Kevin Cummings (Illinois Natural History Survey) • Heidi Dunn, Emily Grossman (Ecological Specialists, Inc.) • Dr. Paul Johnson, Jeff Garner, Michael Buntin, Todd Fobian, Ashley Peters (Alabama Department of Conservation and Natural Resources) • Gerry Dinkins (University of Tennessee) • Matt Johnson, Greg Zimmermann (EnviroScience, Inc.) • Mike Compton, Ian Horn (Kentucky State Nature Preserves Commission) • Chad Lewis, Clarissa Lawliss (Lewis Environmental Consulting) • Nevin Welte (Pennsylvania Boat and Fish Commission) • Amy Mahar, Nick Conrad (New York Natural Heritage Program) • Darran Crabtree (New York Chapter, The Nature Conservancy) • Debbie Wolschki (Ohio Natural Heritage Program) • Janet Clayton (West Virginia Natural Resources) • Brant Fisher (Indiana Department of Natural Resources) • Dr. Monte McGregor, Adam Shepard, Keith Wethington (Kentucky Department of Fish and Wildlife Resources) • Stuart McGregor (Geological Survey of Alabama) • Don Hubbs, Jason Wisenwski (Tennessee Wildlife Resources Agency) • Dr. -
Alabama Charles Lydeard University of Alabama
View metadata, citation and similar papers at core.ac.uk brought to you by CORE provided by Aquila Digital Community Gulf of Mexico Science Volume 17 Article 9 Number 2 Number 2 1999 Freshwater Mussels in the Gulf Region: Alabama Charles Lydeard University of Alabama Jeffrey T. Garner Alabama Game and Fish Division Paul Hartfield U.S. Fish and Wildlife Service James D. Williams U.S. Geological Survey DOI: 10.18785/goms.1702.09 Follow this and additional works at: https://aquila.usm.edu/goms Recommended Citation Lydeard, C., J. T. Garner, P. Hartfield and J. D. Williams. 1999. Freshwater Mussels in the Gulf Region: Alabama. Gulf of Mexico Science 17 (2). Retrieved from https://aquila.usm.edu/goms/vol17/iss2/9 This Article is brought to you for free and open access by The Aquila Digital Community. It has been accepted for inclusion in Gulf of Mexico Science by an authorized editor of The Aquila Digital Community. For more information, please contact [email protected]. Lydeard et al.: Freshwater Mussels in the Gulf Region: Alabama SHORT PAPERS AND NOTES 125 focus on gaming a better understanding of been introduced into the waters of North Amer overall mussel genetics as well as examine the ica. All of these species compete with native problems or benefits of mixing gene pools that mussels for food and space. The Asiatic clam is have been isolated for less than a century. An well established in the southeastern United other potential problem that should be inves States. Though the Asiatic clam can reach den tigated is flooding populations with specimens sities of several hundred per square meter, na propagated from too few individuals. -
Alabama Inventory List
Alabama Inventory List The Rare, Threatened, & Endangered Plants & Animals of Alabama June 2004 Table of Contents INTRODUCTION .....................................................................................................................................................................1 DEFINITION OF HERITAGE RANKS .................................................................................................................................3 DEFINITIONS OF FEDERAL & STATE LISTED SPECIES STATUS.............................................................................5 AMPHIBIANS............................................................................................................................................................................6 BIRDS .........................................................................................................................................................................................7 MAMMALS...............................................................................................................................................................................10 FISHES.....................................................................................................................................................................................12 REPTILES ................................................................................................................................................................................16 CLAMS & MUSSELS ..............................................................................................................................................................18 -
Seasonal and Species-Specific Patterns in Abundance of Freshwater Mussel Glochidia in Stream Drift Author(S): J
Seasonal and species-specific patterns in abundance of freshwater mussel glochidia in stream drift Author(s): J. Jacob Culp, Wendell R. Haag, D. Albrey Arrington, and Thomas B. Kennedy Source: Journal of the North American Benthological Society, 30(2):436-445. 2011. Published By: North American Benthological Society URL: http://www.bioone.org/doi/full/10.1899/10-143.1 BioOne (www.bioone.org) is an electronic aggregator of bioscience research content, and the online home to over 160 journals and books published by not-for-profit societies, associations, museums, institutions, and presses. Your use of this PDF, the BioOne Web site, and all posted and associated content indicates your acceptance of BioOne’s Terms of Use, available at www.bioone.org/page/terms_of_use. Usage of BioOne content is strictly limited to personal, educational, and non-commercial use. Commercial inquiries or rights and permissions requests should be directed to the individual publisher as copyright holder. BioOne sees sustainable scholarly publishing as an inherently collaborative enterprise connecting authors, nonprofit publishers, academic institutions, research libraries, and research funders in the common goal of maximizing access to critical research. J. N. Am. Benthol. Soc., 2011, 30(2):436–445 ’ 2011 by The North American Benthological Society DOI: 10.1899/10-143.1 Published online: 15 March 2011 Seasonal and species-specific patterns in abundance of freshwater mussel glochidia in stream drift 1,4 2,5 3,6 J. Jacob Culp , Wendell R. Haag , D. Albrey Arrington , AND Thomas B. Kennedy1,7 1 University of Alabama, Department of Biological Sciences, P.O. Box 870206, Tuscaloosa, Alabama 35487-0206 USA 2 Center for Bottomland Hardwoods Research, US Department of Agriculture Forest Service, 1000 Front Street, Oxford, Mississippi 38655 USA 3 Loxahatchee River District, 2500 Jupiter Park Drive, Jupiter, Florida 33458 USA Abstract. -
Geological Survey of Alabama
GEOLOGICAL SURVEY OF ALABAMA Berry H. (Nick) Tew, Jr. State Geologist ECOSYSTEMS INVESTIGATIONS PROGRAM Patrick E. O’Neil Director RESULTS OF A SURVEY OF THE MUSSEL FAUNA AT SELECTED STATIONS IN THE BLACK WARRIOR RIVER SYSTEM, ALABAMA, 2009-2012 OPEN-FILE REPORT 1301 By Stuart W. McGregor1, E. Anne Wynn1, and Jeffrey T. Garner2 1Geological Survey of Alabama 2Alabama Department of Conservation and Natural Resources Division of Wildlife and Freshwater Fisheries In cooperation with the Alabama Department of Conservation and Natural Resources Division of Wildlife and Freshwater Fisheries Tuscaloosa, Alabama 2013 CONTENTS Page Abstract............................................................................................................................ 1 Introduction...................................................................................................................... 1 Acknowledgments............................................................................................................ 4 Study area......................................................................................................................... 4 Methods............................................................................................................................ 5 Results and discussion ..................................................................................................... 6 Main channel..................................................................................................... 15 Lower Black Warrior Hydrologic