Information to Users
Total Page:16
File Type:pdf, Size:1020Kb
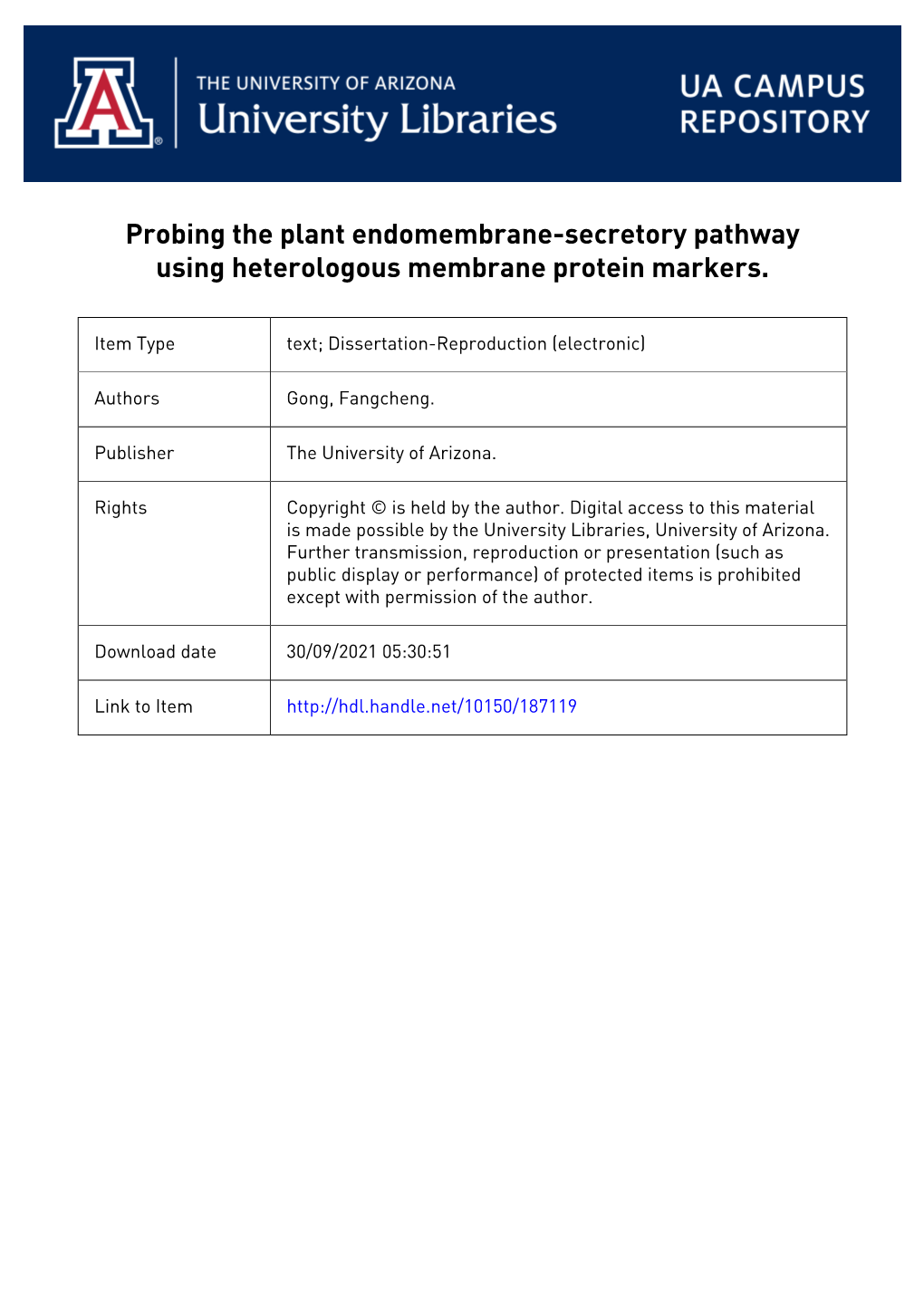
Load more
Recommended publications
-
Characterization of the Gene for the Microbody (Glycosomal) Triosephosphate Isomerase of Trypanosoma Brucei
The EMBO Journal vol.5 no.6 pp. 1291 -1298, 1986 Characterization of the gene for the microbody (glycosomal) triosephosphate isomerase of Trypanosoma brucei Bart W.Swinkels1, Wendy C.Gibson1, Klaas A.Osinga13, isomerase, EC 5.3.1.1) is particularly suitable for such com- Roel Kramer1, Gerrit H.Veeneman2, parative studies. The enzyme is well characterized (see Straus Jacques H.van Boom2 and Piet Borst1 et al., 1985); the amino acid sequence of TIMs from both 'Division of Molecular Biology, The Netherlands Cancer Institute, eukaryotic (Kolb et al., 1974; Corran and Waley, 1975; Alber Plesmanlaan 121, 1066 CX Amsterdam, and 20rganic Chemistry and Kawasaki, 1982; Maquat et al., 1985; Straus and Gilbert, Laboratory, State University Leiden, Gorlaeus Laboratory, PO Box 9502, 1985a) and prokaryotic (Artavanis-Tsakonas and Harris, 1980; 2300 RA Leiden, The Netherlands Pichersky et al., 1984) sources has been determined and high 3Present address: Research and Development, Gist-brocades NV, Postbus 1, resolution structures for the chicken (Banner et al., 1975) and 2600 MA Delft, The Netherlands yeast (Alber et al., 1981) proteins are available. This makes TIM Communicated by P.Borst suitable for deducing long-range evolutionary relationships. To determine how microbody enzymes enter microbodies, we TIM has previously been purified from Trypanosoma brucei are studying the genes for glycosomal (microbody) enzymes (Misset and Opperdoes, 1984) and crystals for X-ray diffraction in Trypanosoma brucei. Here we present our results for triose- have been obtained (Weirenga et al., 1984), allowing the elucida- phosphate isomerase (TIM), which is found exclusively in the tion of the 3-D structure of the enzyme. -
Cell & Molecular Biology
BSC ZO- 102 B. Sc. I YEAR CELL & MOLECULAR BIOLOGY DEPARTMENT OF ZOOLOGY SCHOOL OF SCIENCES UTTARAKHAND OPEN UNIVERSITY BSCZO-102 Cell and Molecular Biology DEPARTMENT OF ZOOLOGY SCHOOL OF SCIENCES UTTARAKHAND OPEN UNIVERSITY Phone No. 05946-261122, 261123 Toll free No. 18001804025 Fax No. 05946-264232, E. mail [email protected] htpp://uou.ac.in Board of Studies and Programme Coordinator Board of Studies Prof. B.D.Joshi Prof. H.C.S.Bisht Retd.Prof. Department of Zoology Department of Zoology DSB Campus, Kumaun University, Gurukul Kangri, University Nainital Haridwar Prof. H.C.Tiwari Dr.N.N.Pandey Retd. Prof. & Principal Senior Scientist, Department of Zoology, Directorate of Coldwater Fisheries MB Govt.PG College (ICAR) Haldwani Nainital. Bhimtal (Nainital). Dr. Shyam S.Kunjwal Department of Zoology School of Sciences, Uttarakhand Open University Programme Coordinator Dr. Shyam S.Kunjwal Department of Zoology School of Sciences, Uttarakhand Open University Haldwani, Nainital Unit writing and Editing Editor Writer Dr.(Ms) Meenu Vats Dr.Mamtesh Kumari , Professor & Head Associate. Professor Department of Zoology, Department of Zoology DAV College,Sector-10 Govt. PG College Chandigarh-160011 Uttarkashi (Uttarakhand) Dr.Sunil Bhandari Asstt. Professor. Department of Zoology BGR Campus Pauri, HNB (Central University) Garhwal. Course Title and Code : Cell and Molecular Biology (BSCZO 102) ISBN : 978-93-85740-54-1 Copyright : Uttarakhand Open University Edition : 2017 Published By : Uttarakhand Open University, Haldwani, Nainital- 263139 Contents Course 1: Cell and Molecular Biology Course code: BSCZO102 Credit: 3 Unit Block and Unit title Page number Number Block 1 Cell Biology or Cytology 1-128 1 Cell Type : History and origin. -
Download (11Mb)
A Thesis Submitted for the Degree of PhD at the University of Warwick Permanent WRAP URL: http://wrap.warwick.ac.uk/108813/ Copyright and reuse: This thesis is made available online and is protected by original copyright. Please scroll down to view the document itself. Please refer to the repository record for this item for information to help you to cite it. Our policy information is available from the repository home page. For more information, please contact the WRAP Team at: [email protected] warwick.ac.uk/lib-publications STUDIES ON THE TARGETING AND PROCESSING OF PRORICIN. Michael Westby BSc. (Hons) Dunelm A thesis submitted for the degree of Doctor of Philosophy Department of Biological Sciences University of Warwick Coventry. U.K. September, 1991 CONTENTS Page No. Contents i-vii List of Figures viii-xi List of Tables xi Acknowledgements xii Declaration xiii Abbreviations xiv-xvi Dedication xvii Summary xviii î.o ihtbodpctioh 1 1.1 Summary 2 1.2 Plant storage proteins 3 1.2.1 Introduction 3 1.2.2 Classification of plant storage proteins 4 1.2.3 Storage proteins of cereals 4 1.2.4 Storage proteins of dicots. 4 1.2.5 Subcellular site of storage protein deposition- the protein bodies 9 1.2.6 Synthesis as preproproteins 11 1.2.7 Summary 15 1.3 Intracellular trafficking of newly-synthesised proteins 16 1.3.1 Introduction 16 1.3.2 Membrane translocation as a first step in compartmentalisation 16 1.3.3 Translocation across the ER membrane 18 1.3.4 Co-translatlonal modifications 21 1.3.5 Protein folding in the ER 21 1.3.6 Protein export -
Protein's Intracellular Adventure Talking About
Dr. Mircea Leabu. The ribosome and intracellular adventure of proteins (lecture iconography) Protein’s intracellular adventure Protein biosynthesis Correct protein folding Non-functional protein degradation Intracellular directing of proteins Talking about Ribosome – structure and function Chaperones – definition and role Proteasome – structure and function Import of proteins into organelles 1 Dr. Mircea Leabu. The ribosome and intracellular adventure of proteins (lecture iconography) Protein biosynthesis •Project – mARN • Machinery – ribosome • Raw material – aminoacyl-tARN Genetic code (degenerated or redundant) Project’s structural organization Eukaryotic messenger ARN 2 Dr. Mircea Leabu. The ribosome and intracellular adventure of proteins (lecture iconography) Raw material structure Eukaryotic transfer ARN (clover leaf) Organization of the machinery Ribosome – 3D Structure opened book lateral view top view Functional3 organization Dr. Mircea Leabu. The ribosome and intracellular adventure of proteins (lecture iconography) Organization of the machinery Molecular organization Biosynthetic process development Stages of protein biosynthesis • Initiation: initiation factors • Elongation: elongation factors • End of translation: releasing factors 4 Dr. Mircea Leabu. The ribosome and intracellular adventure of proteins (lecture iconography) Protein biosynthesis initiation Elongation’s steps Repeating stage, n cycles 5 Dr. Mircea Leabu. The ribosome and intracellular adventure of proteins (lecture iconography) Elongation: energetic needs -
Biol 1020: a Tour of the Cell
Chapter 6: A Tour of the Cell Cell theory Cell organization and homeostasis Studying cells – microscopy and fractionation Eukaryotic vs. prokaryotic cells Compartments in eukaryotic cells (cell regions, organelles) Cytoskeleton Outside the cell . Chapter 6: A Tour of the Cell Cell theory Cell organization and homeostasis Studying cells – microscopy and fractionation Eukaryotic vs. prokaryotic cells Compartments in eukaryotic cells (cell regions, organelles) Cytoskeleton Outside the cell . • What are the main tenets of cell theory? • What are the major lines of evidence that all presently living cells have a common origin? . Cell theory All living organisms are composed of cells smallest “building blocks” of all multicellular organisms all cells are enclosed by a surface membrane that separates them from other cells and from their environment specialized structures with the cell are called organelles; many are membrane-bound . Cell theory Today, all new cells arise from existing cells All presently living cells have a common origin all cells have basic structural and molecular similarities all cells share similar energy conversion reactions all cells maintain and transfer genetic information in DNA the genetic code is essentially universal . • What are the main tenets of cell theory? • What are the major lines of evidence that all presently living cells have a common origin? . Chapter 6: A Tour of the Cell Cell theory Cell organization and homeostasis Studying cells – microscopy and fractionation Eukaryotic vs. prokaryotic cells Compartments in eukaryotic cells (cell regions, organelles) Cytoskeleton Outside the cell . • What is surface area to volume ratio, and why is it an important consideration for cells? • What (usually) happens to surface area to volume ratio as cells grow larger? . -
Structure, Composition, Physical Properties, and Turnover of Proliferated Peroxisomes
STRUCTURE, COMPOSITION, PHYSICAL PROPERTIES, AND TURNOVER OF PROLIFERATED PEROXISOMES A Study of the Trophic Effects of Su-13437 on Rat Liver FEDERICO LEIGHTON, LUCY COLOMA, and CECILIA KOENIG From the Departmento de Biologia Celular, Universidad Catblica de Chile, Santiago, Chile. Dr. Leighton's present address is the International Institute of Cellular and Molecular Pathology, B-1200 Brussels, Belgium. ABSTRACT Peroxisome proliferation has been induced with 2-methyl-2-(p-[l,2,3,4-tetrahy- dro- l-naphthyl]-phenoxy)-propionic acid (Su-13437). DNA, protein, cytochrome oxidase, glucose-6-phosphatase, and acid phosphatase concentrations remain al- most constant. Peroxisomal enzyme activities change to approximately 165%, 50% 30% and 0% of the controls for catalase, urate oxidase, L-a-hydroxy acid oxidase, and D-amino acid oxidase, respectively. For catalase the change results from a decrease in particle-bound activity and a fivefold increase in soluble activ- ity. The average diameter of peroxisome sections is 0.58 • 0.15 tzm in controls and 0.73 • 0.25 ~tm after treatment. Therefore, the measured peroxisomal en- zymes are highly diluted in proliferated particles. After tissue fractionation, approximately one-half of the normal peroxisomes and all proliferated peroxisomes show matric extraction with ghost formation, but no change in size. In homogenates submitted to mechanical stress, proliferated peroxisomes do not reveal increased fragility; unexpectedly, Su-13437 stabilizes lysosomes. Our results suggest that matrix extraction and increased soluble en- zyme activities result from transmembrane passage of peroxisomal proteins. The changes in concentration of peroxisomal oxidases and soluble catalase after Su-13437 allow the calculation of their half-lives. -
Protein Targeting and Degradation 775
Contents CONTENTS CHAPTER • General Considerations • Free and Membrane-bound Ribosomes • Signal Hypothesis • Glycosylation of Proteins at the Level of ER Envelope Carrier Hypothesis 29 • • Proteins with a Carboxyl- terminal KDEL Sequence (=Recycling of Resident Proteins of the ER) Protein • Bacterial Signal Sequences and Protein Targeting • Eukaryotic Protein Transport Targeting and Across Membranes • Protein Import by Receptor- mediated Endocytosis Degradation • Protein Degradation GENERAL CONSIDERATIONS rotein turnover – that is synthesis and degradation – occurs constantly in eukaryotic cells but it is a Phighly selective process with different rates of turnover for various proteins. Turnover of proteins can Nuclear localization signal peptide control the level of certain enzymes, furnish amino acids in times of need and degrade faulty or damaged proteins Protein targeting signal recognition that are generated during synthesis or arise from The structure of the nuclear localization deleterious activities in the cell. Nascent proteins contain signal-binding protein α-karyopherin (or signals that determine their ultimate destination. A newly α-importin) with a nuclear localization signal peptide bound to its major synthesized protein in the prokaryotic Escherichia coli recognition site. cell, for example, can stay in the cytosol or it can be sent to the plasma membrane, the outer membrane, the space between them, or the extracellular medium. The eukaryotic cell is made up of many structures, compartments and organelles, each with specific functions requiring different types of proteins and enzymes. The synthesis of most of these proteins begins on free ribosomes in the cytosol. Therefore, eukaryotic cells must direct proteins to internal sites such as lysosomes, mitochondria, chloroplasts, nucleus etc. How then is sorting accomplished? In eukaryotes, a key choice is made soon after the synthesis of a protein begins. -
Peroxisome Diversity and Evolution
Downloaded from rstb.royalsocietypublishing.org on January 3, 2011 Peroxisome diversity and evolution Toni Gabaldón Phil. Trans. R. Soc. B 2010 365, 765-773 doi: 10.1098/rstb.2009.0240 References This article cites 63 articles, 20 of which can be accessed free http://rstb.royalsocietypublishing.org/content/365/1541/765.full.html#ref-list-1 Rapid response Respond to this article http://rstb.royalsocietypublishing.org/letters/submit/royptb;365/1541/765 Subject collections Articles on similar topics can be found in the following collections cellular biology (98 articles) evolution (2302 articles) Receive free email alerts when new articles cite this article - sign up in the box at the top Email alerting service right-hand corner of the article or click here To subscribe to Phil. Trans. R. Soc. B go to: http://rstb.royalsocietypublishing.org/subscriptions This journal is © 2010 The Royal Society Downloaded from rstb.royalsocietypublishing.org on January 3, 2011 Phil. Trans. R. Soc. B (2010) 365, 765–773 doi:10.1098/rstb.2009.0240 Review Peroxisome diversity and evolution Toni Gabaldo´n* Centre for Genomic Regulation (CRG), Dr Aiguader, 88 08003 Barcelona, Spain Peroxisomes are organelles bounded by a single membrane that can be found in all major groups of eukaryotes. A single evolutionary origin of this cellular compartment is supported by the presence, in diverse organisms, of a common set of proteins implicated in peroxisome biogenesis and maintenance. Their enzymatic content, however, can vary substantially across species, indicating a high level of evol- utionary plasticity. Proteomic analyses have greatly expanded our knowledge on peroxisomes in some model organisms, including plants, mammals and yeasts. -
Establishment of a Fungal Model System for the Study of Ciliation
ESTABLISHMENT OF A FUNGAL MODEL SYSTEM FOR THE STUDY OF CILIATION Linnea Tracy June 2015 ESTABLISHMENT OF A FUNGAL MODEL SYSTEM FOR THE STUDY OF CILIATION An Honors Thesis Submitted to the Department of Biology in partial fulfillment of the Honors Program STANFORD UNIVERSITY by Linnea Tracy June 2015 2 Acknowledgements A simple thank-you seems inadequate for all those who have offered their time, expertise, support, and supplies towards my project and education that is culminating in this thesis. Nevertheless, thank you to Tim Stearns, whose kindness, brilliance, and natural knack for teaching was an inspiration to me as a student, was motivation to me as a researcher, and was a great honor to get to know and work closely with over the last two years. Thank you for your time and dedication devoted to my, my peers’, and the world’s education. A special thank you to Erin Turk, who took me under her wing, learning about chytrids in order to teach and assist me, all the while completing her own graduate dissertation. You are one of the most motivated, prepared, and lovely people I have met at Stanford. Thank you for your guidance, mentorship, and always responding to my text messages. Thank you to the members of the Stearns lab, who guided me from learning the basics of the laboratory through experimental design and science writing. It has been, and continues to be, a great pleasure to have a lab family that is so intelligent and kind. I would be remiss to not also thank my parents and friends, who have loyally allowed me to discuss cilia and worry over my experiments with them, sometimes at the expense of our social life. -
The Development of Microbodies and Peroxisomal Enzymes in Greening Bean Leaves
THE DEVELOPMENT OF MICROBODIES AND PEROXISOMAL ENZYMES IN GREENING BEAN LEAVES PETER J. GRUBER, WAYNE M. BECKER, and ELDON It. NEWCOMB From the Department of Botany, The University of Wisconsin, Madison, Wisconsin 53706 ABSTRACT The ontogeny of leaf microbodies (peroxisomes) has been followed by (a) fixing primary bean leaves at various stages of greening and examining them ultrastructurally, and (b) homogenizing leaves at the same stages and assaying them for three peroxisomal enzymes. A study employing light-grown seedlings showed that when the leaves are still below ground and achlorophyllous, microbodies are present as small organelles (e.g., 0.3 pm in diameter) associated with endoplasmic reticulum, and that after the leaves have turned green and expanded fully, the microbodies occur as much larger organelles (e.g., 1.5/zm in diameter) associated with chloroplasts. Specific activities of the peroxisomal enzymes in- crease 3- to 10-fold during this period. A second study showed that when etiolated seed- lings are transferred to light, the microbodies do not appear to undergo any immediate morphological change, but that by 7~ h they have attained approximately the size and enzymatic activity possessed by microbodies in the mature primary leaves of light-grown plants. It is concluded from the ultrastructural observations that leaf microbodies form as small particles and gradually develop into larger ones through contributions from smooth portions of endoplasmic reticulum. In certain aspects, the development of peroxisomes appears analogous to that of chloroplasts. The possibility is examined that microbodies in green leaves may be relatively long-lived organelles. INTRODUCTION Microbodies are distinctive organelles which were 5, 52) and those occurring in green leaves of characterized first in liver and kidney cells of angiosperms (14, 16, 47, 48) rank among the mammals (6) and later in Tetrahymena (30). -
Evolutionary Transitions Between States of Structural and Developmental Characters Among the Algal Charophyta (Viridiplantae)
ABSTRACT Title of Thesis: EVOLUTIONARY TRANSITIONS BETWEEN STATES OF STRUCTURAL AND DEVELOPMENTAL CHARACTERS AMONG THE ALGAL CHAROPHYTA (VIRIDIPLANTAE). Jeffrey David Lewandowski, Master of Science, 2004 Thesis Directed By: Associate Profe ssor, Charles F. Delwiche, Cell Biology and Molecular Genetics The Charophyta comprise green plant representatives ranging from familiar complex -bodied land plants to subtle and simple forms of green algae, presumably the closest phylogenetic relatives of land plants. This biological lineage provides a unique opportunity to investigate evolutionary transition series that likely facilitated once -aquatic green plants to colonize and diversify in terrestrial environments. A literature review summarizes fu ndamental structural and developmental transitions observed among the major lineages of algal Charophyta. A phylogenetic framework independent of morphological and ontological data is necessary for testing hypotheses about the evolution of structure and development. Thus, to further elucidate the branching order of the algal Charophyta, new DNA sequence data are used to test conflicting hypotheses regarding the phylogenetic placement of several enigmatic taxa, including the algal charophyte genera Mesosti gma , Chlorokybus, Coleochaete , and Chaetosphaeridium. Additionally, technical notes on developing RNA methods for use in studying algal Charophyta are included. EVOLUTIONARY TRANSITIONS BETWEEN STATES OF STRUCTURAL AND DEVELOPMENTAL CHARACTERS AMONG THE ALGAL CHAROPHYTA (VIRIDIPLANTAE). By Jeffrey David Lewandowski Thesis submitted to the Faculty of the Graduate School of the University of Maryland, College Park, in partial fulfillment of the requirements for the degree of Master of Science 2004 Advisory Committee: Associate Professor Charles F. Delwiche, Chair Professor Todd J. Cooke Assistant Professor Eric S. Haag © Copyright by Jeffrey D. Lewandowski 2004 Preface The following are examples from several of the works that have provided philosophical inspiration for this document. -
On the Origin of Microbodies in Plants
On the Origin of Microbodies in Plants - A New Hypothesis - H. Stabenau, U. Winkler, W. Säftel University of Oldenburg Germany Bibliotheks- und Informationssystem der Universität Oldenburg 1998 Correspondence to: H. Stabenau University of Oldenburg FB Biologie Postfach 2503 D-26111 Oldenburg, Germany Verlag/Druck/ Bibliotheks- und Informationssystem Vertrieb: der Carl von Ossietzky Universität Oldenburg (BIS) - Verlag Postfach 2541, 26015 Oldenburg Tel.: 0441/798-2261, Telefax: 0441/798-4040 e-mail: [email protected] ISBN 3-8142-0648-7 Der Druck erfolgte am 26. 10. 1998 On the Origin of Microbodies in Plants H. Stabenau, U. Winkler, W. Säftel University of Oldenburg, Biology Dept., P.O. Box 2503, D-26111 Oldenburg, Germany Abstract. In Euglena gracilis grown with acetate in the dark, the glyoxysomal marker isocitrate lyase was found not to be located in microbodies but in vacuolar structures newly formed by disintegrating mitochondria during heterotrophic growth. The mitochondrial vacuoles contained also spherical organelles with diameters in the range of 0.2 to 0.5 µm and characteristic structures of microbodies. After isolation of these organelles they could be shown to possess only enzymes of the ß-oxidation pathway like microbodies of other primitive algae. Mitochondria disintegrated also in seeds of cucumber which were germinated for three days thereby forming ER-like structures which frequently were seen in close contact with developing microbodies. Altogether, we conclude from our data that microbodies in plant organisms originate from disintegrating mitochondria. Microbodies are organelles which were detected in 1954 and hence demonstrated to be present in eukaryotes of almost all phylogenetic developmental stages but absent from prokaryotes.