Rhodophyta) Reveals A
Total Page:16
File Type:pdf, Size:1020Kb
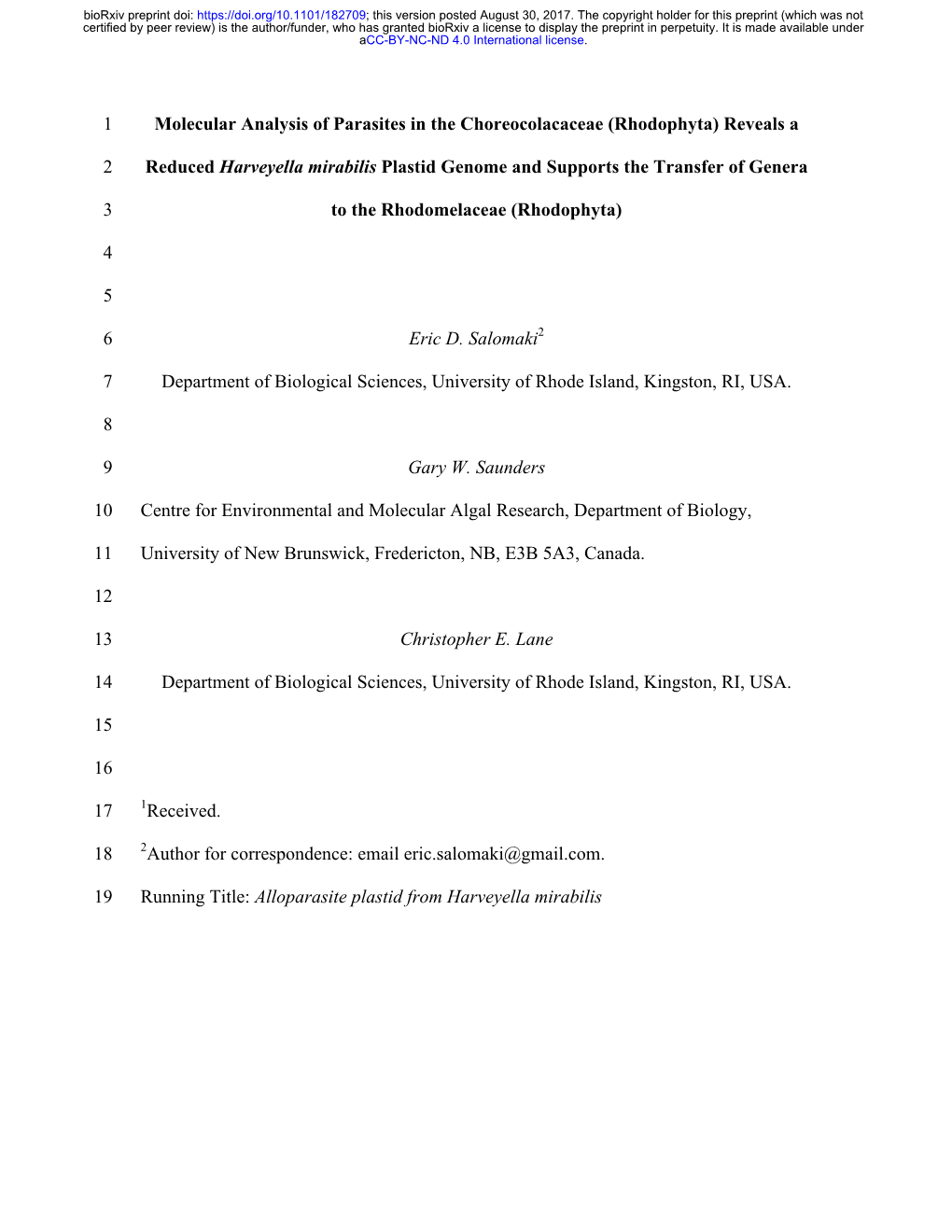
Load more
Recommended publications
-
Newsletter 4
PHYCOLOGICAL NEWSLETTER A PUBLICATION OF THE PHYCOLOGICAL SOCIETY OF AMERICA WINTER INSIDE THIS ISSUE: 2008 PSA Meeting 1 SPRING 2008 Meetings and Symposia 2 Editor: Courses 5 Juan Lopez-Bautista VOLUME 44 Job Opportunities 11 Department of Biological Sciences Trailblazer 28: Sophie C. Ducker 12 University of Alabama Island to honor UAB scientists 18 Tuscaloosa, AL 35487 Books 19 [email protected] Deadline for contributions 23 ∗Dr. Karen Steidinger (Florida Fish and 1 2008 Meeting of Wildlife Research Institute) presenting The Phycological Society of America a plenary talk entitled “Harmful algal blooms in North America: Common risks.” New Orleand, Louisiana, USA NUMBER 27-30 July The associated mini-symposium speakers will be Dr. Leanne Flewelling (Florida Fish he Phycological Society of America (PSA) will and Wildlife Research Institute) present- hold its 2008 annual meeting on July 27-30, ing a talk entitled “Unexpected vectors of 1 T2008 in New Orleans, Louisiana, USA. The brevetoxins to marine mammals” and Dr. meeting will be held on the campus of Loyola Jonathan Deeds (US FDA Center for Food University and is being hosted by Prof. James Wee Safety and Applied Nutrition) present- (Loyola University). The meeting will kick-off with ing a talk entitled “The evolving story of an opening mixer on the evening of Sunday, 27 July Gyrodinium galatheanum = Karlodinium and the scientific program will be Monday through micrum = Karlodinium veneficum. A ten- Wednesday, 28-30 July. The PSA banquet will be year perspective.” Wednesday evening at the Louisiana Swamp Ex- hibit at the Audubon Zoo. Optional field trips are *Dr. John W. -
Habitat Matters for Inorganic Carbon Acquisition in 38 Species Of
View metadata, citation and similar papers at core.ac.uk brought to you by CORE provided by University of Wisconsin-Milwaukee University of Wisconsin Milwaukee UWM Digital Commons Theses and Dissertations August 2013 Habitat Matters for Inorganic Carbon Acquisition in 38 Species of Red Macroalgae (Rhodophyta) from Puget Sound, Washington, USA Maurizio Murru University of Wisconsin-Milwaukee Follow this and additional works at: https://dc.uwm.edu/etd Part of the Ecology and Evolutionary Biology Commons Recommended Citation Murru, Maurizio, "Habitat Matters for Inorganic Carbon Acquisition in 38 Species of Red Macroalgae (Rhodophyta) from Puget Sound, Washington, USA" (2013). Theses and Dissertations. 259. https://dc.uwm.edu/etd/259 This Thesis is brought to you for free and open access by UWM Digital Commons. It has been accepted for inclusion in Theses and Dissertations by an authorized administrator of UWM Digital Commons. For more information, please contact [email protected]. HABITAT MATTERS FOR INORGANIC CARBON ACQUISITION IN 38 SPECIES OF RED MACROALGAE (RHODOPHYTA) FROM PUGET SOUND, WASHINGTON, USA1 by Maurizio Murru A Thesis Submitted in Partial Fulfillment of the Requirements for the Degree of Master of Science in Biological Sciences at The University of Wisconsin-Milwaukee August 2013 ABSTRACT HABITAT MATTERS FOR INORGANIC CARBON ACQUISITION IN 38 SPECIES OF RED MACROALGAE (RHODOPHYTA) FROM PUGET SOUND, WASHINGTON, USA1 by Maurizio Murru The University of Wisconsin-Milwaukee, 2013 Under the Supervision of Professor Craig D. Sandgren, and John A. Berges (Acting) The ability of macroalgae to photosynthetically raise the pH and deplete the inorganic carbon pool from the surrounding medium has been in the past correlated with habitat and growth conditions. -
The Red Alga Polysiphonia (Rhodomelaceae) in the Northern Gulf of California
The Red Alga Polysiphonia (Rhodomelaceae) in the Northern Gulf of California GEORGE J. HOLLENBERG ' • ,. • •a and JAMES N. NORRIS SMITHSONIAN CONTRIBUTIONS TO THE MARINE SCIENCES SERIES PUBLICATIONS OF THE SMITHSONIAN INSTITUTION Emphasis upon publication as a means of "diffusing knowledge" was expressed by the first Secretary of the Smithsonian. In his formal plan for the Institution, Joseph Henry outlined a program that included the following statement: "It is proposed to publish a series of reports, giving an account of the new discoveries in science, and of the changes made from year to year in all branches of knowledge." This theme of basic research has been adhered to through the years by thousands of titles issued in series publications under the Smithsonian imprint, commencing with Smithsonian Contributions to Knowledge in 1848 and continuing with the following active series: Smithsonian Contributions to Anthropology Smithsonian Contributions to Astrophysics Smithsonian Contributions to Botany Smithsonian Contributions to the Earth Sciences Smithsonian Contributions to the Marine Sciences Smithsonian Contributions to Paleobiology Smithsonian Contributions to Zoology Smithsonian Studies in Air and Space Smithsonian Studies in History and Technology In these series, the Institution publishes small papers and full-scale monographs that report the research and collections of its various museums and bureaux or of professional colleagues in the world cf science and scholarship. The publications are distributed by mailing lists to libraries, universities, and similar institutions throughout the world. Papers or monographs submitted for series publication are received by the Smithsonian Institution Press, subject to its own review for format and style, only through departments of the various Smithsonian museums or bureaux, where the manuscripts are given substantive review. -
Algae & Marine Plants of Point Reyes
Algae & Marine Plants of Point Reyes Green Algae or Chlorophyta Genus/Species Common Name Acrosiphonia coalita Green rope, Tangled weed Blidingia minima Blidingia minima var. vexata Dwarf sea hair Bryopsis corticulans Cladophora columbiana Green tuft alga Codium fragile subsp. californicum Sea staghorn Codium setchellii Smooth spongy cushion, Green spongy cushion Trentepohlia aurea Ulva californica Ulva fenestrata Sea lettuce Ulva intestinalis Sea hair, Sea lettuce, Gutweed, Grass kelp Ulva linza Ulva taeniata Urospora sp. Brown Algae or Ochrophyta Genus/Species Common Name Alaria marginata Ribbon kelp, Winged kelp Analipus japonicus Fir branch seaweed, Sea fir Coilodesme californica Dactylosiphon bullosus Desmarestia herbacea Desmarestia latifrons Egregia menziesii Feather boa Fucus distichus Bladderwrack, Rockweed Haplogloia andersonii Anderson's gooey brown Laminaria setchellii Southern stiff-stiped kelp Laminaria sinclairii Leathesia marina Sea cauliflower Melanosiphon intestinalis Twisted sea tubes Nereocystis luetkeana Bull kelp, Bullwhip kelp, Bladder wrack, Edible kelp, Ribbon kelp Pelvetiopsis limitata Petalonia fascia False kelp Petrospongium rugosum Phaeostrophion irregulare Sand-scoured false kelp Pterygophora californica Woody-stemmed kelp, Stalked kelp, Walking kelp Ralfsia sp. Silvetia compressa Rockweed Stephanocystis osmundacea Page 1 of 4 Red Algae or Rhodophyta Genus/Species Common Name Ahnfeltia fastigiata Bushy Ahnfelt's seaweed Ahnfeltiopsis linearis Anisocladella pacifica Bangia sp. Bossiella dichotoma Bossiella -
Acanthophora Dendroides Harvey (Rhodomelaceae), a New Record for the Atlantic and Pacific Oceans
15 3 NOTES ON GEOGRAPHIC DISTRIBUTION Check List 15 (3): 509–514 https://doi.org/10.15560/15.3.509 Acanthophora dendroides Harvey (Rhodomelaceae), a new record for the Atlantic and Pacific oceans Gabriela C. García-Soto, Juan M. Lopez-Bautista The University of Alabama, Department of Biological Sciences, Science and Engineering Complex, 1325 Hackberry Ln, Tuscaloosa, AL 35401, USA. Corresponding author: Gabriela García-Soto, [email protected] Abstract We record Acanthophora dendroides Harvey for the first time in the Atlantic Ocean. Two specimens from the Philip- pines were resolved as conspecific to the Atlantic A. dendroides in molecular analyses extending its geographic range to the Philippines. In light of new evidence provided by field-collected specimens ofAcanthophora spicifera (M.Vahl) Børgesen (generitype) from Florida and Venezuela, the flattened species A. pacifica(Setchell) Kraft, showed no affin- ity to Acanthophora sensu stricto, suggesting that the genus should be restricted to cylindrical species only. Key words Atlantic Ocean, Philippines, taxonomy. Academic editor: Luciane Fontana da Silva | Received 8 October 2018 | Accepted 21 January 2019 | Published 21 June 2019 Citation: García-Soto GC, Lopez-Bautista JM (2019) Acanthophora dendroides Harvey (Rhodomelaceae), a new record for the Atlantic and Pacific oceans. Check List 15 (3): 509–514. https://doi.org/10.15560/15.3.509 Introduction Kraft are restricted to the Pacific Ocean (Guiry and Guiry 2018), A. dendroides Harvey to the Indian Ocean The genus Acanthophora J.V. Lamouroux 1813 is a (Silva et al. 1996) and A. ramulosa Lindenb. ex. Kutz- member of the tribe Chondrieae and it is distinguished from other genera of the tribe by the presence of spirally ing appears to be confined to the Gulf of Guinea in West arranged acute spines (Gordon-Mills and Womersley Africa (Steentoft 1967). -
A Morphological and Phylogenetic Study of the Genus Chondria (Rhodomelaceae, Rhodophyta)
Title A morphological and phylogenetic study of the genus Chondria (Rhodomelaceae, Rhodophyta) Author(s) Sutti, Suttikarn Citation 北海道大学. 博士(理学) 甲第13264号 Issue Date 2018-06-29 DOI 10.14943/doctoral.k13264 Doc URL http://hdl.handle.net/2115/71176 Type theses (doctoral) File Information Suttikarn_Sutti.pdf Instructions for use Hokkaido University Collection of Scholarly and Academic Papers : HUSCAP A morphological and phylogenetic study of the genus Chondria (Rhodomelaceae, Rhodophyta) 【紅藻ヤナギノリ属(フジマツモ科)の形態学的および系統学的研究】 Suttikarn Sutti Department of Natural History Sciences, Graduate School of Science Hokkaido University June 2018 1 CONTENTS Abstract…………………………………………………………………………………….2 Acknowledgement………………………………………………………………………….5 General Introduction………………………………………………………………………..7 Chapter 1. Morphology and molecular phylogeny of the genus Chondria based on Japanese specimens……………………………………………………………………….14 Introduction Materials and Methods Results and Discussions Chapter 2. Neochondria gen. nov., a segregate of Chondria including N. ammophila sp. nov. and N. nidifica comb. nov………………………………………………………...39 Introduction Materials and Methods Results Discussions Conclusion Chapter 3. Yanagi nori—the Japanese Chondria dasyphylla including a new species and a probable new record of Chondria from Japan………………………………………51 Introduction Materials and Methods Results Discussions Conclusion References………………………………………………………………………………...66 Tables and Figures 2 ABSTRACT The red algal tribe Chondrieae F. Schmitz & Falkenberg (Rhodomelaceae, Rhodophyta) currently -
Antimicrobial Activity O Antimicrobial Activity of Marine Algal Extracts E
International Journal of Phytomedicine 9 (2017) 113-122 http://www.arjournals.org/index.php/ijpm/index Original Research Article ISSN: 0975-0185 Antimicrobial activity of marine algal extracts Ekaterina A. Chingizova1*, Anna V. Skriptsova2, Mikhail M. Anisimov1, Dmitry L. Aminin1 *Corresponding author: A b s t r a c t Total, hydrophilic and lipophilic extracts of 21 marine algae species (2 species of Chlorophyta, Ekaterina A. Chingizova 11 of Phaeophyceae and 8 of Rhodophyta) collected along the coast of Russian Far East were screened for antimicrobial activities (against Staphylococcus aureus, Escherichia coli and 1G.B. Elyakov Pacific Institute of Bioorganic Candida albicans). Among the macroalgal extracts analyzed, 95% were active against at least chemistry of the Far-Eastern Branch of one of studied microorganisms, while 80% of extracts were active against two or more test Russian Academy of Sciences , 159 pr. 100 strains. Broad-spectrum activity against three studied microorganisms was observed in the let Vladivostoku, Vladivostok, 690022, 35% of extracts from tested species of marine seaweeds. The results of our survey did not Russia. show clear taxonomic trends in the activities of the total extracts and their hydrophilic fractions 2AV Zhirmunsky Institute of Marine Biology against the studied microorganisms. Nevertheless, lipophilic red algal extracts had both the of the Far-Eastern Branch of Russian highest values and broadest spectrum of bioactivity among all survey algal extracts. More over, Academy of Sciences, 17 Palchevsky Str., lipophilic extracts from red algal exhibited the highest activity against fungus C. albicans Vladivostok, 690041, Russia. among tested algal extracts. Keywords: Antimicrobial activity, extracts, marine algae. -
Ribosomal Dna Phylogeny of the Bangiophycidae (Rhodophyta) and the Origin of Secondary Plastids1
American Journal of Botany 88(8): 1390±1400. 2001. RIBOSOMAL DNA PHYLOGENY OF THE BANGIOPHYCIDAE (RHODOPHYTA) AND THE ORIGIN OF SECONDARY PLASTIDS1 KIRSTEN M. MUÈ LLER,2,6 MARIANA C. OLIVEIRA,3 ROBERT G. SHEATH,2 AND DEBASHISH BHATTACHARYA4,5 2Department of Botany and Dean's Of®ce, University of Guelph, Guelph, Ontario, Canada, N1G 2W1; 3Department of Botany, Institute of Biosciences, University of SaÄo Paulo, R. MataÄo, Travessa 14, N. 321, SaÄo Paulo, SP, Brazil, CEP 05508-900; and 4Department of Biological Sciences, University of Iowa, 239 Biology Building, Iowa City, Iowa 52242-3110 USA We sequenced the nuclear small subunit ribosomal DNA coding region from 20 members of the Bangiophycidae and from two members of the Florideophycidae to gain insights into red algal evolution. A combined alignment of nuclear and plastid small subunit rDNA and a data set of Rubisco protein sequences were also studied to complement the understanding of bangiophyte phylogeny and to address red algal secondary symbiosis. Our results are consistent with a monophyletic origin of the Florideophycidae, which form a sister-group to the Bangiales. Bangiales monophyly is strongly supported, although Porphyra is polyphyletic within Bangia. Ban- giophycidae orders such as the Porphyridiales are distributed over three independent red algal lineages. The Compsopogonales sensu stricto, consisting of two freshwater families, Compsopogonaceae and Boldiaceae, forms a well-supported monophyletic grouping. The single taxon within the Rhodochaetales, Rhodochaete parvula, is positioned within a cluster containing members of the Erythropelti- dales. Analyses of Rubisco sequences show that the plastids of the heterokonts are most closely related to members of the Cyanidiales and are not directly related to cryptophyte and haptophyte plastid genomes. -
Refinements for the Amplification and Sequencing of Red Algal DNA Barcode and Redtol Phylogenetic Markers: a Summary of Current Primers, Profiles and Strategies
Review Algae 2013, 28(1): 31-43 http://dx.doi.org/10.4490/algae.2013.28.1.031 Open Access Refinements for the amplification and sequencing of red algal DNA barcode and RedToL phylogenetic markers: a summary of current primers, profiles and strategies Gary W. Saunders1,* and Tanya E. Moore1 1Centre for Environmental and Molecular Algal Research, Department of Biology, University of New Brunswick, Frederic- ton, NB E3B 5A3, Canada This review provides a comprehensive summary of the PCR primers and profiles currently in use in our laboratory for red algal DNA barcoding and phylogenetic research. While work focuses on florideophyte taxa, many of the markers have been applied successfully to the Bangiales, as well as other lineages previously assigned to the Bangiophyceae sensu lato. All of the primers currently in use with their respective amplification profiles and strategies are provided, which can include full fragment, overlapping fragments and what might best be called “informed overlapping fragments”, i.e., a fragment for a marker is amplified and sequenced for a taxon and those sequence data are then used to identify the best primers to amplify the remaining fragment(s) for that marker. We extend this strategy for the more variable markers with sequence from the external PCR primers used to “inform” the selection of internal sequencing primers. This summary will hopefully serve as a useful resource to systematists in the red algal community. Key Words: DNA barcode; Florideophyceae; molecular markers; phylogeny; polymerase chain reaction; primers; RedToL INTRODUCTION There can be little question that molecular techniques redtol/) (Vis et al. 2010). -
J. Phycol. 53, 32–43 (2017) © 2016 Phycological Society of America DOI: 10.1111/Jpy.12472
J. Phycol. 53, 32–43 (2017) © 2016 Phycological Society of America DOI: 10.1111/jpy.12472 ANALYSIS OF THE COMPLETE PLASTOMES OF THREE SPECIES OF MEMBRANOPTERA (CERAMIALES, RHODOPHYTA) FROM PACIFIC NORTH AMERICA1 Jeffery R. Hughey2 Division of Mathematics, Science, and Engineering, Hartnell College, 411 Central Ave., Salinas, California 93901, USA Max H. Hommersand Department of Biology, University of North Carolina at Chapel Hill, CB# 3280, Coker Hall, Chapel Hill, North Carolina 27599- 3280, USA Paul W. Gabrielson Herbarium and Department of Biology, University of North Carolina at Chapel Hill, CB# 3280, Coker Hall, Chapel Hill, North Carolina 27599-3280, USA Kathy Ann Miller Herbarium, University of California at Berkeley, 1001 Valley Life Sciences Building 2465, Berkeley, California 94720-2465, USA and Timothy Fuller Division of Mathematics, Science, and Engineering, Hartnell College, 411 Central Ave., Salinas, California 93901, USA Next generation sequence data were generated occurring south of Alaska: M. platyphylla, M. tenuis, and used to assemble the complete plastomes of the and M. weeksiae. holotype of Membranoptera weeksiae, the neotype Key index words: Ceramiales; Delesseriaceae; holo- (designated here) of M. tenuis, and a specimen type; Membranoptera; Northeast Pacific; phylogenetic examined by Kylin in making the new combination systematics; plastid genome; plastome; rbcL M. platyphylla. The three plastomes were similar in gene content and length and showed high gene synteny to Calliarthron, Grateloupia, Sporolithon, and Vertebrata. Sequence variation in the plastome Freshwater and Rueness (1994) were the first to coding regions were 0.89% between M. weeksiae and use gene sequences to address species-level taxo- M. tenuis, 5.14% between M. -
The Introduction of the Asian Red Algae Melanothamnus Japonicus
diversity Article The Introduction of the Asian Red Algae Melanothamnus japonicus (Harvey) Díaz-Tapia & Maggs in Peru as a Means to Adopt Management Strategies to Reduce Invasive Non-Indigenous Species Julissa J. Sánchez-Velásquez , Lorenzo E. Reyes-Flores , Carmen Yzásiga-Barrera and Eliana Zelada-Mázmela * Laboratory of Genetics, Physiology, and Reproduction, Faculty of Sciences, Universidad Nacional del Santa, Chimbote 02801, Peru; [email protected] (J.J.S.-V.); [email protected] (L.E.R.-F.); [email protected] (C.Y.-B.) * Correspondence: [email protected] Abstract: Early detection of non-indigenous species is crucial to reduce, mitigate, and manage their impacts on the ecosystems into which they were introduced. However, assessment frameworks for identifying introduced species on the Pacific Coast of South America are scarce and even non-existent for certain countries. In order to identify species’ boundaries and to determine the presence of non-native species, through morphological examinations and the analysis of the plastid ribulose-1,5- Citation: Sánchez-Velásquez, J.J.; rbc Reyes-Flores, L.E.; Yzásiga-Barrera, bisphosphate carboxylase/oxygenase large subunit ( L-5P) gene, we investigated the phylogenetic C.; Zelada-Mázmela, E. The relationships among species of the class Florideophyceae from the coast of Ancash, Peru. The rbcL-5P Introduction of the Asian Red Algae dataset revealed 10 Florideophyceae species distributed in the following four orders: Gigartinales, Melanothamnus japonicus (Harvey) Ceramiales, Halymeniales, and Corallinales, among which the Asian species, Melanothamnus japonicus Díaz-Tapia & Maggs in Peru as a (Harvey) Díaz-Tapia & Maggs was identified. M. japonicus showed a pairwise divergence of 0% Means to Adopt Management with sequences of M. -
Have Elevated Substitution Rates and 12 Extreme Gene Loss in the Plastid Genome 1 13 14
1 2 DR. MAREN PREUSS (Orcid ID : 0000-0002-8147-5643) 3 DR. HEROEN VERBRUGGEN (Orcid ID : 0000-0002-6305-4749) 4 DR. GIUSEPPE C. ZUCCARELLO (Orcid ID : 0000-0003-0028-7227) 5 6 7 Article type : Regular Article 8 9 10 The organelle genomes in the photosynthetic red algal parasite Pterocladiophila 11 hemisphaerica (Florideophyceae, Rhodophyta) have elevated substitution rates and 12 extreme gene loss in the plastid genome 1 13 14 15 Maren Preuss2 16 School of Biological Sciences, Victoria University of Wellington, PO Box 600, Wellington, 17 6140, New Zealand 18 19 Heroen Verbruggen 20 School of BioSciences, University of Melbourne, Parkville, VIC 3010, Australia 21 and 22 Giuseppe C. Zuccarello 23 School of Biological Sciences, Victoria University of Wellington, PO Box 600, Wellington, 24 6140, New Zealand 25 26 27 Author Manuscript 28 Running title: Pterocladiophila organelle genomes 29 This is the author manuscript accepted for publication and has undergone full peer review but has not been through the copyediting, typesetting, pagination and proofreading process, which may lead to differences between this version and the Version of Record. Please cite this article as doi: 10.1111/JPY.12996-20-002 This article is protected by copyright. All rights reserved 30 1 Received Accepted ___________ 31 2 Corresponding author: [email protected] 32 33 34 Editorial Responsibility: M. Coleman (Associate Editor) 35 36 37 ABSTRACT 38 Comparative organelle genome studies of parasites can highlight genetic changes that occur 39 during the transition from a free-living to a parasitic state. Our study focuses on a poorly 40 studied group of red algal parasites, which are often closely related to their red algal hosts and 41 from which they presumably evolved.