A New Vanadium Redox Flow Battery Using Mixed Acid Electrolytes
Total Page:16
File Type:pdf, Size:1020Kb
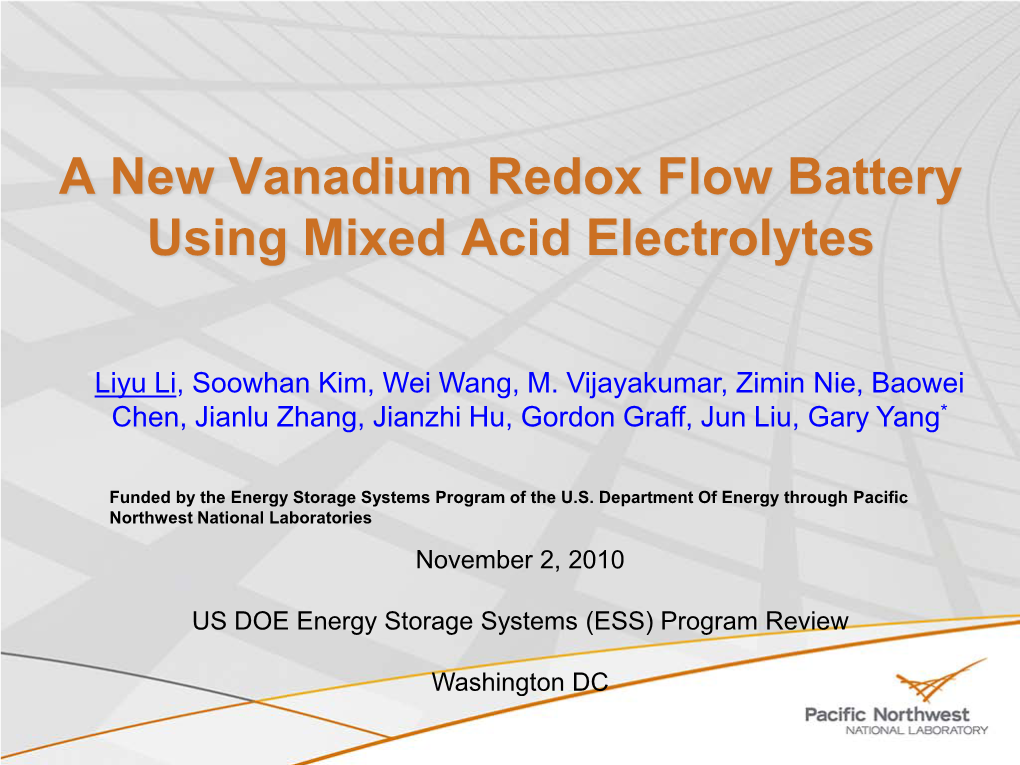
Load more
Recommended publications
-
Characterization of Battery for Energy Storage Applications – Lead Acid
Abstract #1672, 224th ECS Meeting, © 2013 The Electrochemical Society Characterization of battery for energy storage applications curves of a lead acid battery (YUASA, NP4-6). It has a – lead acid battery, lithium battery, vanadium redox normal voltage of 6 V and capacity of 4 Ah. The battery flow battery, and capacitor power was drained at 0.5 A till the cell voltage down to 3.0 V. The E-I curve was recorded by linear scanning Chih-Lung Hsieh1, Yen-Ting Liu2, Kan-Lin Hsueh2, amperometry at scanning rate of 0.01 A/s. The battery is Ju-Shei Hung3 then charge at 0.5 A for 2 hours. The SOC was assumed reached 25%. The E-I curve was then recorded. This 1. Institute of Nuclear Energy Research, Taoyuan, Taiwan procedure was carried out for SOC at 50%, 75%, and 2. Dept. Energy Eng., National United University, Miaoli, 100%. When cell voltage reached 7.0 V during charge Taiwan was considered as 100% SOC. Curves shown on Fig. 2 3. Dept. Chem. Eng., National United University, Miaoli, was measured result of laed acid battery. These curves Taiwan can be described by following equations. Where the IR accounts for internal resistance. Last term of equation [1] is the voltage cahge due to acitvation over-potential. The purpose of this study is to measure the charge/discharge characteristics of four different During Charge components for energy storage application. They are lead RT nF E E 1 iRI o ln o exp[ )]( [1] acid battery, lithium battery, vanadium redox flow battery, nF SO 2 H 4 RT [ 4 [] ] and capacitor. -
Next Generation Anodes for Lithium-Ion Batteries
NEXT GENE ATION ANODES Next Ge eratio A odes for Lithium-Io Batteries Seco d Quarter Progress Report 2018 De is Dees, Poi t-of-Co tact Argonne National Laboratory 9700 South Cass Avenue Argonne, IL 60439 Phone: (630) 252-7349 E-mail: [email protected] Bria Cu i gham, DOE-EERE-VTO Tech ology Ma ager U.S. Department of Energy, Battery R&D Phone: (202) 287-5686 E-mail: [email protected] Table of Co te ts Overview (page 2) 1. Research Facilities Support (page 5) CAMP Facility Support Activities (ANL) (page 5) Characterization and Optimization of Si Slurry by Zeta Potential (ORNL) (page 7) Thermodynamic Understanding and Abuse Performance (SNL) (page 9) Hydro/Solvothermal Synthesis and Scale-up of Silicon and Silicon-containing Nanoparticles (ANL) (page 10) 2. Characterization, Diagnostics, and Analysis (page 12) Spectroscopic Characterization of Cycled Si Electrodes: Understanding the Role of Binder (ORNL) (page 12) EQCM Studies of Silicon Anodes (ANL) (page 13) Effect of silicate thickness on the electrochemical performance of silicate-coated silicon nanoparticles (ANL) (page 15) Calendar-life versus Cycle-life aging of Lithium-ion Cells with Silicon-Graphite Composite Electrodes – Electrolyte Analysis (ANL) (page 17) 3. Materials Advancements (page 18) Continued Study of Lithiation Effect of the Poly(Acrylic Acid) Binders on the Silicon Anode of Lithium-Ion Batteries (ANL) (page 19) Probe the relationships between functional electrolytes structure and SEI property for Si materials (LBNL) (page 21) Silicon Surface Modification Using -
Current State and Future Prospects for Electrochemical Energy Storage and Conversion Systems
energies Review Current State and Future Prospects for Electrochemical Energy Storage and Conversion Systems Qaisar Abbas 1 , Mojtaba Mirzaeian 2,3,*, Michael R.C. Hunt 1, Peter Hall 2 and Rizwan Raza 4 1 Centre for Materials Physics, Department of Physics, Durham University, Durham DH1 3LE, UK; [email protected] (Q.A.); [email protected] (M.R.H.) 2 School of Computing, Engineering and Physical Sciences, University of the West of Scotland, Paisley PA1 2BE, UK; [email protected] 3 Faculty of Chemistry and Chemical Technology, Al-Farabi Kazakh National University, Al-Farabi Avenue, 71, Almaty 050040, Kazakhstan 4 Clean Energy Research Lab (CERL), Department of Physics, COMSATS University Islamabad, Lahore 54000, Pakistan; [email protected] * Correspondence: [email protected] Received: 30 September 2020; Accepted: 26 October 2020; Published: 9 November 2020 Abstract: Electrochemical energy storage and conversion systems such as electrochemical capacitors, batteries and fuel cells are considered as the most important technologies proposing environmentally friendly and sustainable solutions to address rapidly growing global energy demands and environmental concerns. Their commercial applications individually or in combination of two or more devices are based on their distinguishing properties e.g., energy/power densities, cyclability and efficiencies. In this review article, we have discussed some of the major electrochemical energy storage and conversion systems and encapsulated their technological advancement in recent years. Fundamental working principles and material compositions of various components such as electrodes and electrolytes have also been discussed. Furthermore, future challenges and perspectives for the applications of these technologies are discussed. -
Elements of Electrochemistry
Page 1 of 8 Chem 201 Winter 2006 ELEM ENTS OF ELEC TROCHEMIS TRY I. Introduction A. A number of analytical techniques are based upon oxidation-reduction reactions. B. Examples of these techniques would include: 1. Determinations of Keq and oxidation-reduction midpoint potentials. 2. Determination of analytes by oxidation-reductions titrations. 3. Ion-specific electrodes (e.g., pH electrodes, etc.) 4. Gas-sensing probes. 5. Electrogravimetric analysis: oxidizing or reducing analytes to a known product and weighing the amount produced 6. Coulometric analysis: measuring the quantity of electrons required to reduce/oxidize an analyte II. Terminology A. Reduction: the gaining of electrons B. Oxidation: the loss of electrons C. Reducing agent (reductant): species that donates electrons to reduce another reagent. (The reducing agent get oxidized.) D. Oxidizing agent (oxidant): species that accepts electrons to oxidize another species. (The oxidizing agent gets reduced.) E. Oxidation-reduction reaction (redox reaction): a reaction in which electrons are transferred from one reactant to another. 1. For example, the reduction of cerium(IV) by iron(II): Ce4+ + Fe2+ ! Ce3+ + Fe3+ a. The reduction half-reaction is given by: Ce4+ + e- ! Ce3+ b. The oxidation half-reaction is given by: Fe2+ ! e- + Fe3+ 2. The half-reactions are the overall reaction broken down into oxidation and reduction steps. 3. Half-reactions cannot occur independently, but are used conceptually to simplify understanding and balancing the equations. III. Rules for Balancing Oxidation-Reduction Reactions A. Write out half-reaction "skeletons." Page 2 of 8 Chem 201 Winter 2006 + - B. Balance the half-reactions by adding H , OH or H2O as needed, maintaining electrical neutrality. -
All-Carbon Electrodes for Flexible Solar Cells
applied sciences Article All-Carbon Electrodes for Flexible Solar Cells Zexia Zhang 1,2,3 ID , Ruitao Lv 1,2,*, Yi Jia 4, Xin Gan 1,5 ID , Hongwei Zhu 1,2 and Feiyu Kang 1,5,* 1 State Key Laboratory of New Ceramics and Fine Processing, School of Materials Science and Engineering, Tsinghua University, Beijing 100084, China; [email protected] (Z.Z.); [email protected] (X.G.); [email protected] (H.Z.) 2 Key Laboratory of Advanced Materials (MOE), School of Materials Science and Engineering, Tsinghua University, Beijing 100084, China 3 School of Physics and Electronic Engineering, Xinjiang Normal University, Urumqi 830046, Xinjiang Province, China 4 Qian Xuesen Laboratory of Space Technology, China Academy of Space Technology, Beijing 100094, China; [email protected] 5 Graduate School at Shenzhen, Tsinghua University, Shenzhen 518055, Guangdong Province, China * Correspondences: [email protected] (R.L.); [email protected] (F.K.) Received: 16 December 2017; Accepted: 20 January 2018; Published: 23 January 2018 Abstract: Transparent electrodes based on carbon nanomaterials have recently emerged as new alternatives to indium tin oxide (ITO) or noble metal in organic photovoltaics (OPVs) due to their attractive advantages, such as long-term stability, environmental friendliness, high conductivity, and low cost. However, it is still a challenge to apply all-carbon electrodes in OPVs. Here, we report our efforts to develop all-carbon electrodes in organic solar cells fabricated with different carbon-based materials, including carbon nanotubes (CNTs) and graphene films synthesized by chemical vapor deposition (CVD). Flexible and semitransparent solar cells with all-carbon electrodes are successfully fabricated. -
A Review of Cathode and Anode Materials for Lithium-Ion Batteries
A Review of Cathode and Anode Materials for Lithium-Ion Batteries Yemeserach Mekonnen Aditya Sundararajan Arif I. Sarwat IEEE Student Member IEEE Student Member IEEE Member Department of Electrical & Department of Electrical & Department of Electrical & Computer Engineering Computer Engineering Computer Engineering Florida International University Florida International University Florida International University Email: [email protected] Email: [email protected] Email: [email protected] Abstract—Lithium ion batteries are one of the most technologies such as plug-in HEVs. For greater application use, commercially sought after energy storages today. Their batteries are usually expensive and heavy. Li-ion and Li- based application widely spans from Electric Vehicle (EV) to portable batteries show promising advantages in creating smaller, devices. Their lightness and high energy density makes them lighter and cheaper battery storage for such high-end commercially viable. More research is being conducted to better applications [18]. As a result, these batteries are widely used in select the materials for the anode and cathode parts of Lithium (Li) ion cell. This paper presents a comprehensive review of the common consumer electronics and account for higher sale existing and potential developments in the materials used for the worldwide [2]. Lithium, as the most electropositive element making of the best cathodes, anodes and electrolytes for the Li- and the lightest metal, is a unique element for the design of ion batteries such that maximum efficiency can be tapped. higher density energy storage systems. The discovery of Observed challenges in selecting the right set of materials is also different inorganic compounds that react with alkali metals in a described in detail. -
Recent Progress in Vanadium Redox-Flow Battery Katsuji Emura1 Sumitomo Electric Industries, Ltd., Osaka, Japan
Recent Progress in Vanadium Redox-Flow Battery Katsuji Emura1 Sumitomo Electric Industries, Ltd., Osaka, Japan 1. Introduction Vanadium Redox Flow Battery (VRB) is an energy storage system that employs a rechargeable vanadium fuel cell technology. Since 1985, Sumitomo Electric Industries Ltd (SEI) has developed VRB technologies for large-scale energy storage in collaboration with Kansai Electric power Co. In 2001, SEI has developed 3MW VRB system and delivered it to a large liquid crystal display manufacturing plant. The VRB system provides 3MW of power for 1.5 seconds as UPS (Uninterruptible Power Supply) for voltage sag compensation and 1.5MW of power for 1hr as a peak shaver to reduce peak load. The VRB system has successfully compensated for 50 voltage sag events that have occurred since installation up until September 2003. 2. Principle of VRB The unique chemistry of vanadium allows it to be used in both the positive and negative electrolytes. Figure 1 shows a schematic configuration of VRB system. The liquid electrolytes are circulated through the fuel cells in a similar manner to that of hydrogen and oxygen in a hydrogen fuel cell. Similarly, the electrochemical reactions occurring within the cells can produce a current flow in an external circuit, alternatively reversing the current flow results in recharging of the electrolytes. These cell reactions are balanced by a flow of protons or hydrogen ions across the cell through a selective membrane. The selective membrane also serves as a physical barrier keeping the positive and negative vanadium electrolytes separate. Figure 2 shows a cell stack which was manufactured by SEI. -
A Highly-Active, Stable and Low-Cost Platinum-Free Anode Catalyst Based on Runi for Hydroxide Exchange Membrane Fuel Cells
ARTICLE https://doi.org/10.1038/s41467-020-19413-5 OPEN A highly-active, stable and low-cost platinum-free anode catalyst based on RuNi for hydroxide exchange membrane fuel cells Yanrong Xue1,2,5, Lin Shi3,5, Xuerui Liu1,2, Jinjie Fang1,2, Xingdong Wang2, Brian P. Setzler3, Wei Zhu 2, ✉ ✉ Yushan Yan 1,3 & Zhongbin Zhuang 1,2,4 1234567890():,; The development of cost-effective hydroxide exchange membrane fuel cells is limited by the lack of high-performance and low-cost anode hydrogen oxidation reaction catalysts. Here we report a Pt-free catalyst Ru7Ni3/C, which exhibits excellent hydrogen oxidation reaction activity in both rotating disk electrode and membrane electrode assembly measurements. The hydrogen oxidation reaction mass activity and specific activity of Ru7Ni3/C, as measured in rotating disk experiments, is about 21 and 25 times that of Pt/C, and 3 and 5 times that of PtRu/C, respectively. The hydroxide exchange membrane fuel cell with Ru7Ni3/C anode can −2 −2 deliver a high peak power density of 2.03 W cm in H2/O2 and 1.23 W cm in H2/air (CO2-free) at 95 °C, surpassing that using PtRu/C anode catalyst, and good durability with less than 5% voltage loss over 100 h of operation. The weakened hydrogen binding of Ru by alloying with Ni and enhanced water adsorption by the presence of surface Ni oxides lead to the high hydrogen oxidation reaction activity of Ru7Ni3/C. By using the Ru7Ni3/C catalyst, the anode cost can be reduced by 85% of the current state-of-the-art PtRu/C, making it highly promising in economical hydroxide exchange membrane fuel cells. -
Galvanic Cell Notation • Half-Cell Notation • Types of Electrodes • Cell
Galvanic Cell Notation ¾Inactive (inert) electrodes – not involved in the electrode half-reaction (inert solid conductors; • Half-cell notation serve as a contact between the – Different phases are separated by vertical lines solution and the external el. circuit) 3+ 2+ – Species in the same phase are separated by Example: Pt electrode in Fe /Fe soln. commas Fe3+ + e- → Fe2+ (as reduction) • Types of electrodes Notation: Fe3+, Fe2+Pt(s) ¾Active electrodes – involved in the electrode ¾Electrodes involving metals and their half-reaction (most metal electrodes) slightly soluble salts Example: Zn2+/Zn metal electrode Example: Ag/AgCl electrode Zn(s) → Zn2+ + 2e- (as oxidation) AgCl(s) + e- → Ag(s) + Cl- (as reduction) Notation: Zn(s)Zn2+ Notation: Cl-AgCl(s)Ag(s) ¾Electrodes involving gases – a gas is bubbled Example: A combination of the Zn(s)Zn2+ and over an inert electrode Fe3+, Fe2+Pt(s) half-cells leads to: Example: H2 gas over Pt electrode + - H2(g) → 2H + 2e (as oxidation) + Notation: Pt(s)H2(g)H • Cell notation – The anode half-cell is written on the left of the cathode half-cell Zn(s) → Zn2+ + 2e- (anode, oxidation) + – The electrodes appear on the far left (anode) and Fe3+ + e- → Fe2+ (×2) (cathode, reduction) far right (cathode) of the notation Zn(s) + 2Fe3+ → Zn2+ + 2Fe2+ – Salt bridges are represented by double vertical lines ⇒ Zn(s)Zn2+ || Fe3+, Fe2+Pt(s) 1 + Example: A combination of the Pt(s)H2(g)H Example: Write the cell reaction and the cell and Cl-AgCl(s)Ag(s) half-cells leads to: notation for a cell consisting of a graphite cathode - 2+ Note: The immersed in an acidic solution of MnO4 and Mn 4+ reactants in the and a graphite anode immersed in a solution of Sn 2+ overall reaction are and Sn . -
Demonstrating Solar Conversion Using Natural Dye Sensitizers
Demonstrating Solar Conversion Using Natural Dye Sensitizers Subject Area(s) Science & Technology, Physical Science, Environmental Science, Physics, Biology, and Chemistry Associated Unit Renewable Energy Lesson Title Dye Sensitized Solar Cell (DSSC) Grade Level (11th-12th) Time Required 3 hours / 3 day lab Summary Students will analyze the use of solar energy, explore future trends in solar, and demonstrate electron transfer by constructing a dye-sensitized solar cell using vegetable and fruit products. Students will analyze how energy is measured and test power output from their solar cells. Engineering Connection and Tennessee Careers An important aspect of building solar technology is the study of the type of materials that conduct electricity and understanding the reason why they conduct electricity. Within the TN-SCORE program Chemical Engineers, Biologist, Physicist, and Chemists are working together to provide innovative ways for sustainable improvements in solar energy technologies. The lab for this lesson is designed so that students apply their scientific discoveries in solar design. Students will explore how designing efficient and cost effective solar panels and fuel cells will respond to the social, political, and economic needs of society today. Teachers can use the Metropolitan Policy Program Guide “Sizing The Clean Economy: State of Tennessee” for information on Clean Economy Job Growth, TN Clean Economy Profile, and Clean Economy Employers. www.brookings.edu/metro/clean_economy.aspx Keywords Photosynthesis, power, electricity, renewable energy, solar cells, photovoltaic (PV), chlorophyll, dye sensitized solar cells (DSSC) Page 1 of 10 Next Generation Science Standards HS.ESS-Climate Change and Human Sustainability HS.PS-Chemical Reactions, Energy, Forces and Energy, and Nuclear Processes HS.ETS-Engineering Design HS.ETS-ETSS- Links Among Engineering, Technology, Science, and Society Pre-Requisite Knowledge Vocabulary: Catalyst- A substance that increases the rate of reaction without being consumed in the reaction. -
Nickel-Metal Hydride Batteries: a New Generation of Secondary Storage
8 Nickel-Metal Hydride Batteries: A New Generation of Secondary Storage Brian Niece Literature Seminar September 23, 1993 Interest in secondary batteries suitable for use in electric vehicles has increased rapidly in the last decade, as restrictions on vehicle emissions in urban areas have become more stringent. Combustion of fossil fuels in large power plants and storage of the energy electrochemically is more efficient than direct combustion in internal combustion engines [1]. In addition, storage batteries can be charged from alternative sources of electricity, such as solar and hydroelectric plants. In order to be acceptable for use in an electric vehicle, a battery must meet a number of important criteria. It must have a high energy density to make the travel distance between recharges as long as possible. It must be capable of high power output throughout its dis charge cycle to provide the high current necessary to operate a vehicle, and it must be capa ble of especially high peak power for acceleration. The battery must also have a long cycle life as well as a long physical lifetime. It must not undergo rapid self discharge on standing. Finally, it must not be prohibitively expensive. Theoretical energy densities of secondary battery systems in common use are listed in Table 1. Most of these systems are not practical for electric vehicle use for a variety of rea sons. The lead-sulfuric acid battery cannot be sealed, and cannot be produced with a suffi ciently high energy density. The sodium-sulfur cell is undesirable because it contains metal lic sodium and operates at 300°C. -
Chapter 13: Electrochemical Cells
March 19, 2015 Chapter 13: Electrochemical Cells electrochemical cell: any device that converts chemical energy into electrical energy, or vice versa March 19, 2015 March 19, 2015 Voltaic Cell -any device that uses a redox reaction to transform chemical potential energy into electrical energy (moving electrons) -the oxidizing agent and reducing agent are separated -each is contained in a half cell There are two half cells in a voltaic cell Cathode Anode -contains the SOA -contains the SRA -reduction reaction -oxidation takes place takes place - (-) electrode -+ electrode -anions migrate -cations migrate towards the anode towards cathode March 19, 2015 Electrons move through an external circuit from the anode to cathode Electricity is produced by the cell until one of the reactants is used up Example: A simple voltaic cell March 19, 2015 When designing half cells it is important to note the following: -each half cell needs an electrolyte and a solid conductor -the electrode and electrolyte cannot react spontaneously with each other (sometimes carbon and platinum are used as inert electrodes) March 19, 2015 There are two kinds of porous boundaries 1. Salt Bridge 2. Porous Cup · an unglazed ceramic cup · tube filled with an inert · separates solutions but electrolyte such as NaNO allows ions to pass 3 through or Na2SO4 · the ends are plugged so the solutions are separated, but ions can pass through Porous boundaries allow for ions to move between two half cells so that charge can be equalized between two half cells 2+ 2– electrolyte: Cu (aq), SO4 (aq) 2+ 2– electrolyte: Zn (aq), SO4 (aq) electrode: zinc electrode: copper March 19, 2015 Example: Metal/Ion Voltaic Cell V Co(s) Zn(s) Co2+ SO 2- 4 2+ SO 2- Zn 4 Example: A voltaic cell with an inert electrode March 19, 2015 Example Label the cathode, anode, electron movement, ion movement, and write the half reactions taking place at each half cell.