Tunable Plasmonic Metamaterials for Subwavelength Focusing
Total Page:16
File Type:pdf, Size:1020Kb
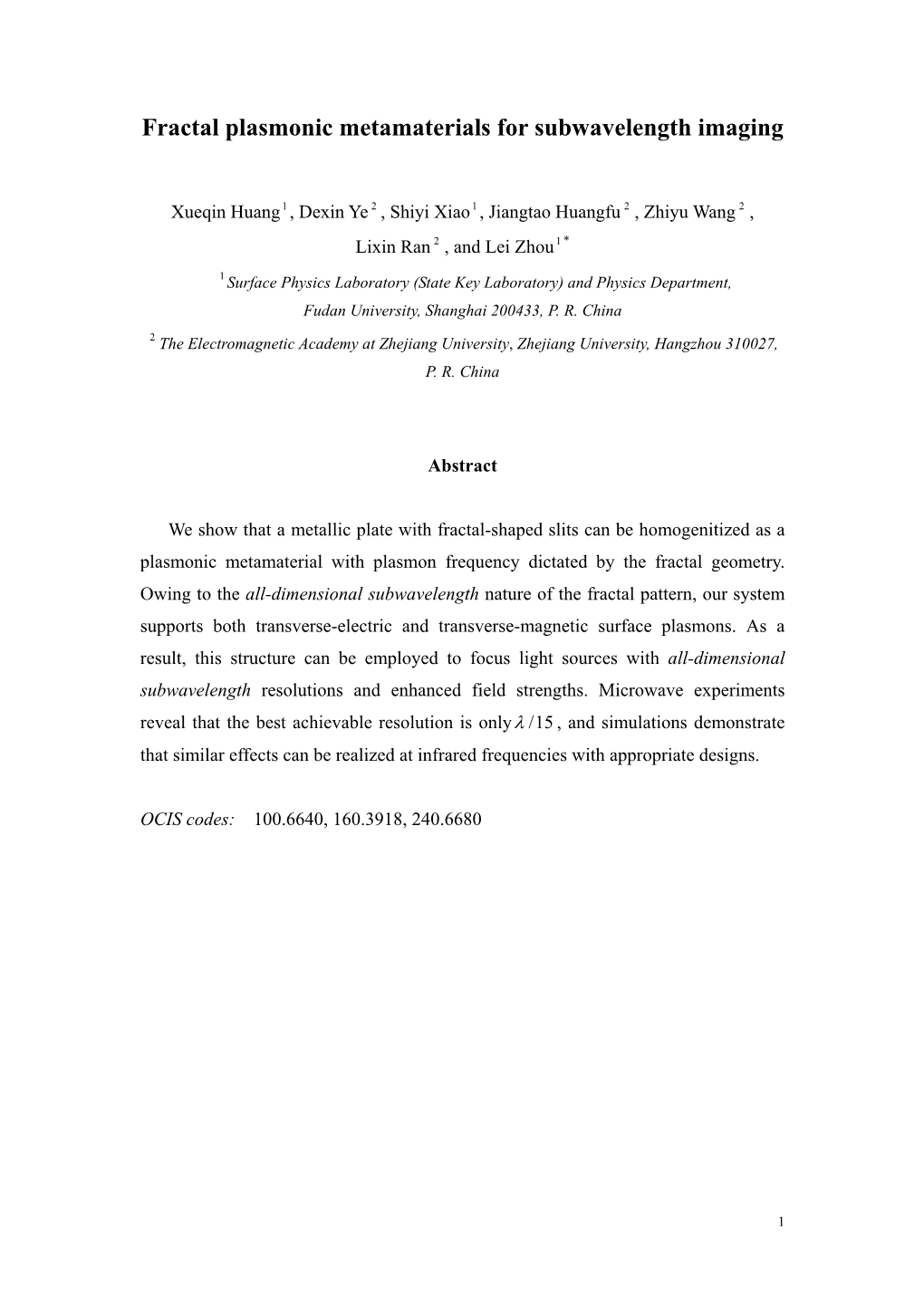
Load more
Recommended publications
-
Plasmonic and Metamaterial Structures As Electromagnetic Absorbers
Plasmonic and Metamaterial Structures as Electromagnetic Absorbers Yanxia Cui 1,2, Yingran He1, Yi Jin1, Fei Ding1, Liu Yang1, Yuqian Ye3, Shoumin Zhong1, Yinyue Lin2, Sailing He1,* 1 State Key Laboratory of Modern Optical Instrumentation, Centre for Optical and Electromagnetic Research, Zhejiang University, Hangzhou 310058, China 2 Key Lab of Advanced Transducers and Intelligent Control System, Ministry of Education and Shanxi Province, College of Physics and Optoelectronics, Taiyuan University of Technology, Taiyuan, 030024, China 3 Department of Physics, Hangzhou Normal University, Hangzhou 310012, China Corresponding author: e-mail [email protected] Abstract: Electromagnetic absorbers have drawn increasing attention in many areas. A series of plasmonic and metamaterial structures can work as efficient narrow band absorbers due to the excitation of plasmonic or photonic resonances, providing a great potential for applications in designing selective thermal emitters, bio-sensing, etc. In other applications such as solar energy harvesting and photonic detection, the bandwidth of light absorbers is required to be quite broad. Under such a background, a variety of mechanisms of broadband/multiband absorption have been proposed, such as mixing multiple resonances together, exciting phase resonances, slowing down light by anisotropic metamaterials, employing high loss materials and so on. 1. Introduction physical phenomena associated with planar or localized SPPs [13,14]. Electromagnetic (EM) wave absorbers are devices in Metamaterials are artificial assemblies of structured which the incident radiation at the operating wavelengths elements of subwavelength size (i.e., much smaller than can be efficiently absorbed, and then transformed into the wavelength of the incident waves) [15]. They are often ohmic heat or other forms of energy. -
Enhancing the Resolution of Imaging Systems by Spatial Spectrum Manipulation
Michigan Technological University Digital Commons @ Michigan Tech Dissertations, Master's Theses and Master's Reports 2019 Enhancing the Resolution of Imaging Systems by Spatial Spectrum Manipulation Wyatt Adams Michigan Technological University, [email protected] Copyright 2019 Wyatt Adams Recommended Citation Adams, Wyatt, "Enhancing the Resolution of Imaging Systems by Spatial Spectrum Manipulation", Open Access Dissertation, Michigan Technological University, 2019. https://doi.org/10.37099/mtu.dc.etdr/861 Follow this and additional works at: https://digitalcommons.mtu.edu/etdr Part of the Electromagnetics and Photonics Commons ENHANCING THE RESOLUTION OF IMAGING SYSTEMS BY SPATIAL SPECTRUM MANIPULATION By Wyatt Adams A DISSERTATION Submitted in partial fulfillment of the requirements for the degree of DOCTOR OF PHILOSOPHY In Electrical Engineering MICHIGAN TECHNOLOGICAL UNIVERSITY 2019 © 2019 Wyatt Adams This dissertation has been approved in partial fulfillment of the requirements for the Degree of DOCTOR OF PHILOSOPHY in Electrical Engineering. Department of Electrical and Computer Engineering Dissertation Advisor: Dr. Durdu G¨uney Committee Member: Dr. Paul Bergstrom Committee Member: Dr. Christopher Middlebrook Committee Member: Dr. Miguel Levy Department Chair: Dr. Glen Archer Dedication To my parents for their love, guidance, and wisdom. Contents Preface ...................................... xi Acknowledgments ............................... xv Abstract ..................................... xvii 1 Introduction ................................ -
Metamaterials
Tie Jun Cui • David R. Smith • Ruopeng Liu Editors Metamaterials Theory, Design, and Applications Springer Contents 1 Introduction to Metamatenals 1 Tie Jun Cui, Ruopeng Liu and David R. Smith 1.1 What Is Metamaterial? 1 1.2 From Left-Handed Material to Invisible Cloak: A Brief History... 4 1.3 Optical Transformation and Control of Electromagnetic Waves ... 5 1.4 Homogenization of Artificial Particles and Effective Medium Theory 6 1.4.1 General Description 6 1.4.2 A TL-Metamaterial Example 8 1.5 Rapid Design of Metamatenals 14 1.6 Resonant and Non-resonant Metamatenals 14 1.7 Applications of Metamatenals 16 1.8 Computational Electromagnetics: A New Aspect of Metamatenals 16 References , 17 2 Optical Transformation Theory 21 Wei Xiang Jiang and Tie Jun Cui 2.1 Introduction 21 2.2 Optical Transformation Medium 22 2.3 Transformation Devices 25 2.3.1 Invisibility Cloaks 25 2.3.2 EM Concentrators 33 2.3.3 EM-Field and Polarization Rotators 35 2.3.4 Wave-Shape Transformers 36 2.3.5 EM-Wave Bending 37 2.3.6 More Invisibility Devices 39 2.3.7 Other Optical-Transformation Devices 41 2.4 Summary 43 References 44 3 General Theory on Artificial Metamatenals 49 Ruopeng Liu, Tie Jun Cui and David R. Smith 3.1 Local Field Response and Spatial Dispersion Effect on Metamatenals 50 XIII XIV Contents 3.2 Spatial Dispersion Model on Artificial Metamaterials 53 3.3 Explanation of the Behavior on Metamaterial Structures 55 3.4 Verification of the Spatial Dispersion Model 56 References 58 4 Rapid Design for Metamaterials 61 Jessie Y. -
Metamaterials for Photonic Applications Natalia Dubrovina
Metamaterials for photonic applications Natalia Dubrovina To cite this version: Natalia Dubrovina. Metamaterials for photonic applications. Other [cond-mat.other]. Université Paris Sud - Paris XI, 2014. English. NNT : 2014PA112088. tel-01522399 HAL Id: tel-01522399 https://tel.archives-ouvertes.fr/tel-01522399 Submitted on 15 May 2017 HAL is a multi-disciplinary open access L’archive ouverte pluridisciplinaire HAL, est archive for the deposit and dissemination of sci- destinée au dépôt et à la diffusion de documents entific research documents, whether they are pub- scientifiques de niveau recherche, publiés ou non, lished or not. The documents may come from émanant des établissements d’enseignement et de teaching and research institutions in France or recherche français ou étrangers, des laboratoires abroad, or from public or private research centers. publics ou privés. UNIVERSITE PARIS-SUD ECOLE DOCTORALE : Ondes et M atiere Institut d’Electronique Fondamentale (IEF) DISCIPLINE PHYSIQUE THÈSE DE DOCTORAT Soutenue le 14.05.2014 par Natalia Dubrovina M etamaterials for photonic applications Directeur de thèse : Anatole LUPU Chargé de recherche CNRS, Insititut d’Electronique Fondamentale Composition du jury : Président du jury : André DE LUSTRAC Professeur, Université Paris Ouest Rapporteurs : Andrei LAVRINENKO Professeur, Technical University of Denmark Yannick DE WILDE Directeur de recherche, Institut Langevin, ESPCI-ParisTech Examinateurs : Alexandre BOUHELIER Chargé de recherche, Institut Carnot de Bourgogne Anatoly ZAYATS Professeur, King's College London this thesis I dedicate to the memory of my father, V yatcheslav !"#$%& " !%&'( $)"&* )%+ &,$, -".% '$!$ Acknowledgments This thesis would not have been written without number of people to whom I am deeply thankful and to whom I would like to express my greatest gratitude. -
Spoof Surface Plasmon Polaritons Supported by Ultrathin Corrugated Metal Strip and Their Applications
Nanotechnol Rev 2015; 4(3): 239–258 Review Xi Gao and Tie Jun Cui* Spoof surface plasmon polaritons supported by ultrathin corrugated metal strip and their applications Abstract: In this review, we present a brief introduction Attributing to remarkable features and huge application on the spoof surface plasmons supported on corrugated potentials [3–10], SPPs have attracted extensive attentions metallic plates with nearly zero thickness. We mainly and have been intensively investigated. At optical frequen- focus on the propagation characteristics of spoof surface cies, metals behave like plasmas with negative permittiv- plasmon polaritons (SPPs), excitation of planar SPPs, and ity, which makes SPPs be highly confined to the interface several plasmonic devices including the bending wave- of metal and air (or metal and dielectric) and propagate guide, Y-shaped beam splitter, frequency splitter, and fil- along the surface. SPPs can overcome diffraction limit ter. These devices are designed and fabricated with either and realize miniaturized photonic components and inte- planar or conformal plasmonic metamaterials, which are grated circuits due to their highly localized feature, which validated by both full-wave simulations and experiments, makes it widely used in nano-photonics and optoelectron- showing high performance. We also demonstrate that an ics [4, 11–14]. However, as the frequency goes downward to ultrathin textured metallic disk can support multipolar microwave and terahertz regions, the natural SPPs do not spoof localized surface plasmons, either with straight or exist on smooth metal surfaces because of infinite dielec- curved grooves, from which the Fano resonances are also tric constant of metal [1]. Instead, Sommerfeld or Zenneck observed. -
76362252.Pdf
University of Pennsylvania ScholarlyCommons Departmental Papers (ESE) Department of Electrical & Systems Engineering June 2007 Cloaking and transparency for collections of particles with metamaterial and plasmonic covers Andrea Alù University of Pennsylvania Nader Engheta University of Pennsylvania, [email protected] Follow this and additional works at: http://repository.upenn.edu/ese_papers Recommended Citation Andrea Alù and Nader Engheta, "Cloaking and transparency for collections of particles with metamaterial and plasmonic covers", . June 2007. Copyright 2007 Optical Society of America, Inc. Postprint version. Published in Optics Express, Volume 16, Issue 12, June 11, 2007, pages 7578-7590. Publisher URL: http://www.opticsexpress.org/Issue.cfm This paper is posted at ScholarlyCommons. http://repository.upenn.edu/ese_papers/257 For more information, please contact [email protected]. Cloaking and transparency for collections of particles with metamaterial and plasmonic covers Abstract Following our recently developed idea of employing plasmonic covers to cloak an isolated conducting, plasmonic or insulating sphere through scattering cancellation, here we extend this concept by investigating the possibility of cloaking multiple objects placed in close proximity of each other, or even joined together to form a single object of large electrical size. We show how the coupling among the single particles, even when placed in the very near zone of each other, is drastically lowered by the presence of suitably designed covers, thus providing the possibility of making collections of objects transparent and "cloaked" to the impinging radiation even when the total physical size of the system is sensibly larger than the wavelength. Numerical simulations and animations validate these results and give further insights into the anomalous phenomenon of transparency and cloaking induced by plasmonic materials and metamaterials. -
Integrated Spoof Plasmonic Circuits ⇑ Jingjing Zhang, Hao-Chi Zhang, Xin-Xin Gao, Le-Peng Zhang, Ling-Yun Niu, Pei-Hang He, Tie-Jun Cui
Science Bulletin 64 (2019) 843–855 Contents lists available at ScienceDirect Science Bulletin journal homepage: www.elsevier.com/locate/scib Review Integrated spoof plasmonic circuits ⇑ Jingjing Zhang, Hao-Chi Zhang, Xin-Xin Gao, Le-Peng Zhang, Ling-Yun Niu, Pei-Hang He, Tie-Jun Cui State Key Laboratory of Millimeter Waves, Southeast University, Nanjing 210096, China article info abstract Article history: Using a metamaterial consisting of metals with subwavelength surface patterning, one can mimic surface Received 30 November 2018 plasmon polaritons (SPPs) and achieve surface waves with subwavelength confinement at microwave Received in revised form 21 January 2019 and terahertz frequencies, thus bringing most of the advantages associated with the optical SPPs to lower Accepted 23 January 2019 frequencies. Due to the properties of strong field confinement and high local field intensity, spoof SPPs Available online 2 February 2019 have demonstrated the improved performance for data transmission and device miniaturization in an intensively integrated environment. The distinctive abilities, such as suppression of transmission loss Keywords: and bending loss, and increase of signal integrity, make spoof SPPs a promising candidate for future gen- Spoof surface plasmons eration of electronic circuits and electromagnetic systems. This article reviews the progress in spoof SPPs Metamaterial Electromagnetic system with a special focus on their applications in circuits from transmission lines to passive and active devices Integrated circuits in microwave and terahertz regimes. The integration of versatile spoof SPP devices on a single platform, which is compatible with established electronic circuits, is also discussed. Ó 2019 Science China Press. Published by Elsevier B.V. and Science China Press. -
Optical Measurements on Plasmonic Metamaterials
Optical Measurements on Plasmonic Metamaterials Ruben C. Maas Supervisors: Dr. James Parsons and Prof. Dr. Albert Polman Research project for the masters degree in Nanomaterials: Chemistry and Physics at Utrecht University, The Netherlands August 2010 - June 2011 Center for Nanophotonics FOM Institute AMOLF Amsterdam, The Netherlands Abstract Metamaterials are unique in their ability to control the confinement and propagation of light, exhibiting electromagnetic phenomena not observed in conventional materials. In this work, analytical calculations are used to design a plasmonic metamaterial with a three dimensional negative index of refraction at optical frequencies. A brief overview of the theory describing these metamaterials is presented. Samples are fabricated using electron beam physical vapor deposition. Variable angle spectroscopic ellipsometry is used to determine the optical constants and thicknesses of the evaporated layers. In addition, Rutherford backscattering spectrometry, optical transmission and reflection measurements and a focus ion beam created cross-section are used to analyze multilayer stacks. From these multilayer stacks miniature prisms are sculpted. A Fourier microscope which specifically operates at UV and visible frequencies is con- structed to perform refraction measurements on the miniature prisms. The effective refrac- tive index is derived from the refraction angle using Snell's law. Experimental measurements show good agreement with finite-difference time-domain simulations, but do not show a neg- ative refractive index. The observed effective index is explained using an effective medium theory. Contents 1 Introduction 3 1.1 Metamaterials and light . .3 2 Plasmonic Metamaterials 6 2.1 Metal dielectric interfaces . .6 2.2 Plasmonic waveguides . .7 2.3 Metamaterials and mode index . -
Mxenes for Plasmonic and Metamaterial Devices
FM2G.7.pdf CLEO 2018 © OSA 2018 MXenes for Plasmonic and Metamaterial Devices Zhuoxian Wang,1 Krishnakali Chaudhuri,1 Mohamed Alhabeb,2 Xiangeng Meng,1 Shaimaa I Azzam,1 Alexander Kildishev,1 Young L. Kim,3 Vladimir M. Shalaev,1 Yury Gogotsi, 2 and Alexandra Boltasseva1 1School of Electrical and Computer Engineering, and Birck Nanotechnology Center, Purdue University, West Lafayette, IN 47907, USA 2Department of Materials Science and Engineering, and A. J. Drexel Nanomaterials Institute, Drexel University, Philadelphia, PA 19104, USA 3Weldon School of Biomedical Engineering, Purdue University, West Lafayette, IN 47907, USA Author e-mail address: [email protected] Abstract We explore the applications of MXenes, a new material class of growing interest, in the area of nanophotonics and plasmonics. A broadband plasmonic metamaterial absorber and a random laser device have thus been demonstrated. OCIS codes: (160.3918) Metamaterials; (250.5403) Plasmonics; (140.3460) Lasers 1. Introduction MXenes are a new family of two-dimensional (2D) nanomaterials formed of transition metal carbides and carbon nitrides that shows high metallic conductivity, surface hydrophilicity and excellent mechanical properties [1-2]. They are derived from layered ternary carbides and nitrides known as MAX (Mn+1AXn) phases by selective chemical etching of the ‘A’ layers and addition of surface functional groups ‘T’ (=O, -OH or -F), making the final composition of Mn+1XnTx [3]. MXenes have been explored in a variety of applications [3]; however, investigations of MXenes in the context of nanophotonics and plasmonics have been limited [4-5]. This motivates the current study of MXenes as building blocks for plasmonic and metamaterial devices. -
Christos Argyropoulos
CHRISTOS ARGYROPOULOS CONTACT DETAILS Assistant Professor, Department of Electrical Engineering, University of Nebraska-Lincoln, SEC, Room 239N, 844 N. 16th St, Lincoln, NE, 68588-0511, USA. Email: [email protected] Alt. Email: [email protected] Website: http://argyropoulos.unl.edu/ Tel: +1 402-472-3710 RESEARCH INTERESTS Linear and nonlinear plasmonics and nanophotonics, metamaterials and their applications, antenna design, transformation electromagnetics, photonics, active, tunable and reconfigurable metadevices, acoustic/thermal metamaterials, microwave/mm-wave/THz engineering, novel optical interconnects, thermal emission from plasmonic structures, graphene nanophotonics, novel energy harvesting devices and computational electromagnetics. EDUCATION 2007-2011 PhD in Electronic Engineering Thesis Project: FDTD Modelling of Electromagnetic Transformation Based Devices Queen Mary, University of London, Department of Electronic Engineering Antennas & Electromagnetics Group, London, UK Supervisors: Prof. Yang Hao, Prof. Clive Parini 2006-2007 MSc in Communication Engineering Dissertation Project: An approach to measure the input impedance and the far- field distribution of an antenna system with the FDTD method The University of Manchester, School of Electrical and Electronic Engineering Microwaves and Communication Systems Group, Manchester, UK Supervisors: Dr. Fumie Costen, Prof. Tony Brown 2000-2006 Diploma in Electrical and Computer Engineering Diploma Thesis: Fast computation of scattering cross section of thin coatings over metallic surfaces Aristotle University of Thessaloniki, School of Technology, Faculty of Electrical and Computer Engineering, Department of Telecommunications, Thessaloniki, Greece Supervisor: Dr. Traianos V. Yioultsis HONORS, AWARDS, ACTIVITIES June-Oct 2015 Presented lecture titled 'Nano Light Technology' at the 2015 Nebraska Center for Materials and Nanoscience (NCMN) annual ‘Bright Lights NanoCamp’ and at the NCMN ‘After School’ program to an audience of underrepresented middle school students. -
Using Plasmonic Cloaking Method on in Nite Cylindrical Structures and Its
Using Plasmonic Cloaking Method on Innite Cylindrical Structures and its Applications Afsaneh Rezaei Shiraz University Farzad Mohajeri ( [email protected] ) Shiraz University https://orcid.org/0000-0002-0444-7614 Zahra Hamzavi Zarghani Shiraz University Research Article Keywords: Plasmonic, Metamaterial, Plasmonic cloak, Reverse polarization Posted Date: June 9th, 2021 DOI: https://doi.org/10.21203/rs.3.rs-494808/v1 License: This work is licensed under a Creative Commons Attribution 4.0 International License. Read Full License Using Plasmonic Cloaking Method on Infinite Cylindrical Structures and its Applications Afsaneh Rezaei1, Farzad Mohajeri2,*, Zahra Hamzavi-Zarghani3 1 M.Sc., Department of Communications and Electronics, School of Electrical and Computer Engineering, Shiraz University, Shiraz, Iran. 2 Associate Professor, Department of Communications and Electronics, School of Electrical and Computer Engineering, Shiraz University, Shiraz, Iran. 3 Ph.D., Department of Communications and Electronics, School of Electrical and Computer Engineering, Shiraz University, Shiraz, Iran. *Corresponding author. E-mail: [email protected] (A.Rezaei), [email protected] (F.Mohajeri), [email protected] (Z.Hamzavi-Zarghani). Abstract: In recent years, cloaking using materials with negative electric permittivity or magnetic permeability has been studied and researched. It has been demonstrated that covering an object with a cloak that has negative electric permittivity or magnetic permeability or less than one can cause a reduction of the scattering cross section (SCS) of the object. In this paper, we have solved the problem of scattering for the object and single-layer or multi-layer cylindrical cloaks and by doing so, we have obtained the necessary fundamental equations for designing these types of cloaks in two conditions, with and without considering the effects of coupling on the mathematics of solving the problem of scattering. -
Plasmonic Metamaterial Gels with Spatially Patterned Orientational Order Via 3D Printing † † † ∥ ‡ ‡ ⊥ Andrew J
This is an open access article published under an ACS AuthorChoice License, which permits copying and redistribution of the article or any adaptations for non-commercial purposes. Article Cite This: ACS Omega 2019, 4, 20558−20563 http://pubs.acs.org/journal/acsodf Plasmonic Metamaterial Gels with Spatially Patterned Orientational Order via 3D Printing † † † ∥ ‡ ‡ ⊥ Andrew J. Hess, Andrew J. Funk, Qingkun Liu, , Joshua A. De La Cruz, Ghadah H. Sheetah, , † † ‡ § Blaise Fleury, and Ivan I. Smalyukh*, , , † Department of Physics, 390 UCB, University of Colorado Boulder, Boulder, Colorado 80309, United States ‡ Materials Science and Engineering, 027 UCB, Sustainability, Energy & Environment Community, University of Colorado Boulder, Boulder, Colorado 80303, United States § Renewable and Sustainable Energy Institute, National Renewable Energy Laboratory and University of Colorado, Boulder, Colorado 80309, United States *S Supporting Information ABSTRACT: Optical properties can be programmed on mesoscopic scales by patterning host materials while ordering their nanoparticle inclusions. While liquid crystals are often used to define the ordering of nanoparticles dispersed within them, this approach is typically limited to liquid crystals confined in classic geometries. In this work, the orientational order that liquid crystalline colloidal hosts impose on anisotropic nanoparticle inclusions is combined with an additive manufacturing method that enables engineered, macroscopic three-dimensional (3D) patterns of co-aligned gold nanorods and cellulose