RESEARCH ARTICLES the Complete
Total Page:16
File Type:pdf, Size:1020Kb
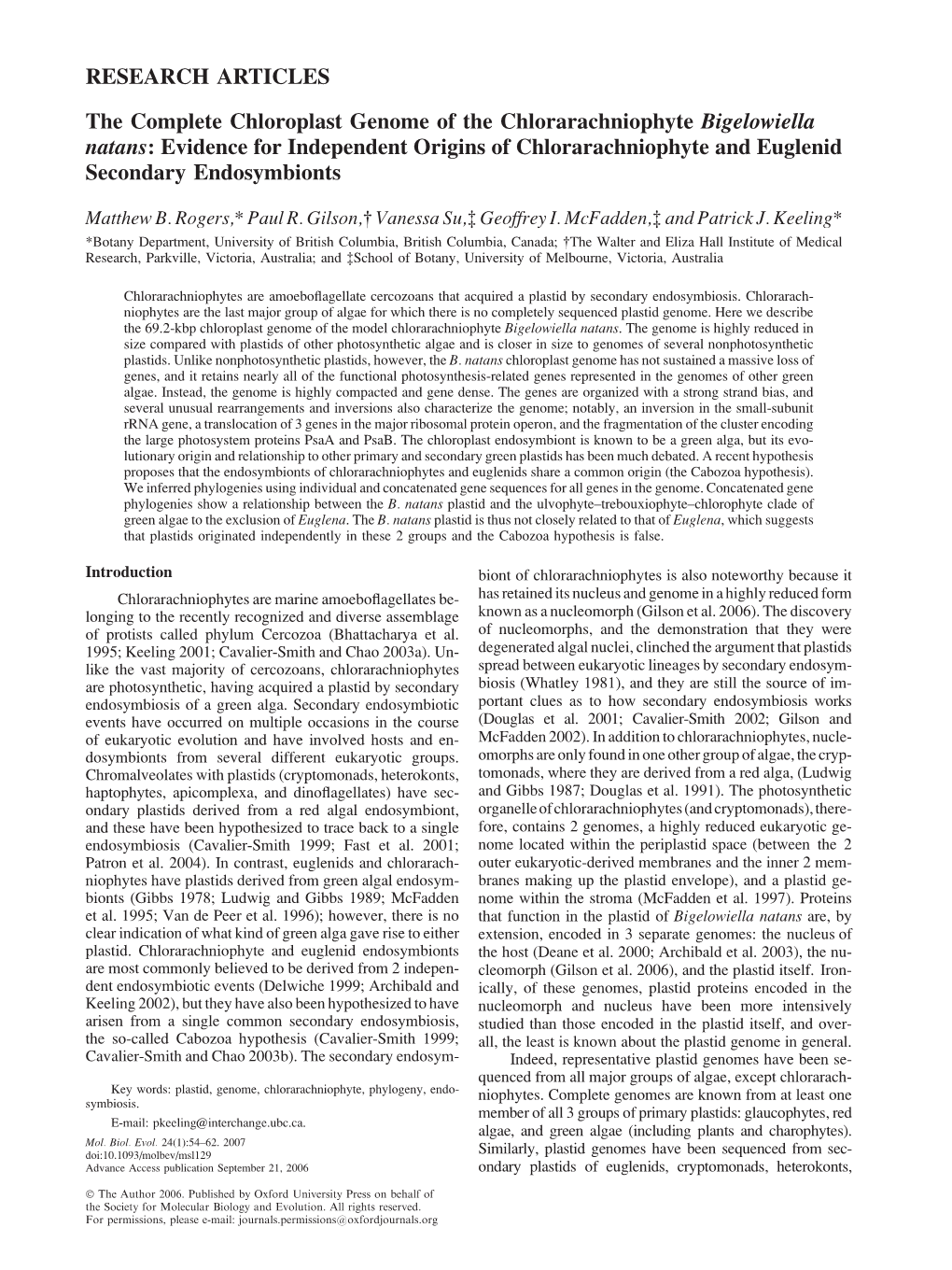
Load more
Recommended publications
-
Molecular Data and the Evolutionary History of Dinoflagellates by Juan Fernando Saldarriaga Echavarria Diplom, Ruprecht-Karls-Un
Molecular data and the evolutionary history of dinoflagellates by Juan Fernando Saldarriaga Echavarria Diplom, Ruprecht-Karls-Universitat Heidelberg, 1993 A THESIS SUBMITTED IN PARTIAL FULFILMENT OF THE REQUIREMENTS FOR THE DEGREE OF DOCTOR OF PHILOSOPHY in THE FACULTY OF GRADUATE STUDIES Department of Botany We accept this thesis as conforming to the required standard THE UNIVERSITY OF BRITISH COLUMBIA November 2003 © Juan Fernando Saldarriaga Echavarria, 2003 ABSTRACT New sequences of ribosomal and protein genes were combined with available morphological and paleontological data to produce a phylogenetic framework for dinoflagellates. The evolutionary history of some of the major morphological features of the group was then investigated in the light of that framework. Phylogenetic trees of dinoflagellates based on the small subunit ribosomal RNA gene (SSU) are generally poorly resolved but include many well- supported clades, and while combined analyses of SSU and LSU (large subunit ribosomal RNA) improve the support for several nodes, they are still generally unsatisfactory. Protein-gene based trees lack the degree of species representation necessary for meaningful in-group phylogenetic analyses, but do provide important insights to the phylogenetic position of dinoflagellates as a whole and on the identity of their close relatives. Molecular data agree with paleontology in suggesting an early evolutionary radiation of the group, but whereas paleontological data include only taxa with fossilizable cysts, the new data examined here establish that this radiation event included all dinokaryotic lineages, including athecate forms. Plastids were lost and replaced many times in dinoflagellates, a situation entirely unique for this group. Histones could well have been lost earlier in the lineage than previously assumed. -
Norrisiella Sphaerica Gen
Norrisiella sphaerica gen. et sp. nov., a new coccoid chlorarachniophyte from Baja California, Mexico 著者 Ota Shuhei, Ueda Kunihiko, Ishida Ken-ichiro journal or Journal of Plant Research publication title volume 120 number 6 page range 661-670 year 2007-11-01 URL http://hdl.handle.net/2297/7674 doi: 10.1007/s10265-007-0115-y Norrisiella sphaerica gen. et sp. nov., a new coccoid chlorarachniophyte from Baja California, Mexico Shuhei Ota1, 2, Kunihiko Ueda1 and Ken-ichiro Ishida2 1Division of Life Sciences, Graduate School of Natural Science and Technology, Kanazawa University, Kakuma, Kanazawa 920-1192, Japan 2Graduate School of Life and Environmental Sciences, University of Tsukuba, 1-1-1 Tennodai, Tsukuba 305-8572, Japan Running title: Norrisiella sphaerica gen. et sp. nov. Correspondence: Shuhei Ota Laboratory of Plant Systematics and Phylogeny (D508) Institute of Biological Sciences Graduate School of Life and Environmental Sciences University of Tsukuba, 1-1-1, Tennodai, Tsukuba 305-8572, Japan Tel/Fax: +81 (29) 853-7267 Fax: +81 (29) 853-4533 e-mail: [email protected] 1/29 Abstract A new chlorarachniophyte, Norrisiella sphaerica S. Ota et K. Ishida gen. et sp. nov., is described from the coast of Baja California, Mexico. We examined its morphology, ultrastructure and life cycle in detail, using light microscopy, transmission electron microscopy and time-lapse videomicroscopy. We found that this chlorarachniophyte possessed the following characteristics: (i) vegetative cells were coccoid and possessed a cell wall, (ii) a pyrenoid was slightly invaded by plate-like periplastidial compartment from the tip of the pyrenoid, (iii) a nucleomorph was located near the pyrenoid base in the periplastidial compartment, (iv) cells reproduced vegetatively via autospores, and (v) a flagellate stage was present in the life cycle. -
23.3 Groups of Protists
Chapter 23 | Protists 639 cysts that are a protective, resting stage. Depending on habitat of the species, the cysts may be particularly resistant to temperature extremes, desiccation, or low pH. This strategy allows certain protists to “wait out” stressors until their environment becomes more favorable for survival or until they are carried (such as by wind, water, or transport on a larger organism) to a different environment, because cysts exhibit virtually no cellular metabolism. Protist life cycles range from simple to extremely elaborate. Certain parasitic protists have complicated life cycles and must infect different host species at different developmental stages to complete their life cycle. Some protists are unicellular in the haploid form and multicellular in the diploid form, a strategy employed by animals. Other protists have multicellular stages in both haploid and diploid forms, a strategy called alternation of generations, analogous to that used by plants. Habitats Nearly all protists exist in some type of aquatic environment, including freshwater and marine environments, damp soil, and even snow. Several protist species are parasites that infect animals or plants. A few protist species live on dead organisms or their wastes, and contribute to their decay. 23.3 | Groups of Protists By the end of this section, you will be able to do the following: • Describe representative protist organisms from each of the six presently recognized supergroups of eukaryotes • Identify the evolutionary relationships of plants, animals, and fungi within the six presently recognized supergroups of eukaryotes • Identify defining features of protists in each of the six supergroups of eukaryotes. In the span of several decades, the Kingdom Protista has been disassembled because sequence analyses have revealed new genetic (and therefore evolutionary) relationships among these eukaryotes. -
Gymnochlora Dimorpha Sp. Nov., a Chlorarachniophyte with Unique Daughter Cell Behaviour
Phycologia (2011) Volume 50 (3), 317–326 Published 3 May 2011 Gymnochlora dimorpha sp. nov., a chlorarachniophyte with unique daughter cell behaviour 1,2 3 1 SHUHEI OTA *{,ASTUKO KUDO AND KEN-ICHIRO ISHIDA 1Institute of Biological Sciences, Graduate School of Life and Environmental Sciences, University of Tsukuba, 1-1-1, Tennodai, Tsukuba 305-8572, Japan 2Station Biologique de Roscoff, Universite´ Pierre et Marie Curie (Paris 06), CNRS and UMR 7144, Place Georges Tessier, 29682 Roscoff, France 3The College of Biological Sciences, University of Tsukuba, 1-1-1, Tennodai, Tsukuba 305-8572, Japan OTA S., KUDO A. AND ISHIDA K.-I. 2011. Gymnochlora dimorpha sp. nov., a chlorarachniophyte with unique daughter cell behaviour. Phycologia 50: 317–326. DOI: 10.2216/09-102.1 A new chlorarachniophyte species, Gymnochlora dimorpha sp. nov., was described. This new species was isolated from an enrichment preculture of Padina sp. collected from a subtidal coral reef zone in Republic of Palau. The new strain, P314, was characterized by light and electron microscopy in the present study. Under the culture conditions used here, the amoeboid stage was dominant. Two types of amoeboid cells were found in the cultures: motile and flattened nonmotile (sessile) cells. The motile cells typically multiplied via binary cell division. The sessile cells were always present in the cultures, but they never became dominant under the culture conditions. Time-lapse video microscopic observations revealed that after cell division of a sessile cell, one daughter cell became motile, while the other remained sessile. According to ultrastructural characteristics of the pyrenoids and nucleomorphs, the new chlorarachniophyte strain belongs to the genus Gymnochlora. -
VII EUROPEAN CONGRESS of PROTISTOLOGY in Partnership with the INTERNATIONAL SOCIETY of PROTISTOLOGISTS (VII ECOP - ISOP Joint Meeting)
See discussions, stats, and author profiles for this publication at: https://www.researchgate.net/publication/283484592 FINAL PROGRAMME AND ABSTRACTS BOOK - VII EUROPEAN CONGRESS OF PROTISTOLOGY in partnership with THE INTERNATIONAL SOCIETY OF PROTISTOLOGISTS (VII ECOP - ISOP Joint Meeting) Conference Paper · September 2015 CITATIONS READS 0 620 1 author: Aurelio Serrano Institute of Plant Biochemistry and Photosynthesis, Joint Center CSIC-Univ. of Seville, Spain 157 PUBLICATIONS 1,824 CITATIONS SEE PROFILE Some of the authors of this publication are also working on these related projects: Use Tetrahymena as a model stress study View project Characterization of true-branching cyanobacteria from geothermal sites and hot springs of Costa Rica View project All content following this page was uploaded by Aurelio Serrano on 04 November 2015. The user has requested enhancement of the downloaded file. VII ECOP - ISOP Joint Meeting / 1 Content VII ECOP - ISOP Joint Meeting ORGANIZING COMMITTEES / 3 WELCOME ADDRESS / 4 CONGRESS USEFUL / 5 INFORMATION SOCIAL PROGRAMME / 12 CITY OF SEVILLE / 14 PROGRAMME OVERVIEW / 18 CONGRESS PROGRAMME / 19 Opening Ceremony / 19 Plenary Lectures / 19 Symposia and Workshops / 20 Special Sessions - Oral Presentations / 35 by PhD Students and Young Postdocts General Oral Sessions / 37 Poster Sessions / 42 ABSTRACTS / 57 Plenary Lectures / 57 Oral Presentations / 66 Posters / 231 AUTHOR INDEX / 423 ACKNOWLEDGMENTS-CREDITS / 429 President of the Organizing Committee Secretary of the Organizing Committee Dr. Aurelio Serrano -
Single Cell Transcriptomics, Mega-Phylogeny and the Genetic Basis Of
bioRxiv preprint doi: https://doi.org/10.1101/064030; this version posted July 15, 2016. The copyright holder for this preprint (which was not certified by peer review) is the author/funder, who has granted bioRxiv a license to display the preprint in perpetuity. It is made available under aCC-BY 4.0 International license. 1 Single cell transcriptomics, mega-phylogeny and the genetic basis of 2 morphological innovations in Rhizaria 3 4 Anders K. Krabberød1, Russell J. S. Orr1, Jon Bråte1, Tom Kristensen1, Kjell R. Bjørklund2 & Kamran 5 ShalChian-Tabrizi1* 6 7 1Department of BiosCienCes, Centre for Integrative MiCrobial Evolution and Centre for EpigenetiCs, 8 Development and Evolution, University of Oslo, Norway 9 2Natural History Museum, Department of ResearCh and ColleCtions University of Oslo, Norway 10 11 *Corresponding author: 12 Kamran ShalChian-Tabrizi 13 [email protected] 14 Mobile: + 47 41045328 15 16 17 Keywords: Cytoskeleton, phylogeny, protists, Radiolaria, Rhizaria, SAR, single-cell, transCriptomiCs 1 bioRxiv preprint doi: https://doi.org/10.1101/064030; this version posted July 15, 2016. The copyright holder for this preprint (which was not certified by peer review) is the author/funder, who has granted bioRxiv a license to display the preprint in perpetuity. It is made available under aCC-BY 4.0 International license. 18 Abstract 19 The innovation of the eukaryote Cytoskeleton enabled phagoCytosis, intracellular transport and 20 Cytokinesis, and is responsible for diverse eukaryotiC morphologies. Still, the relationship between 21 phenotypiC innovations in the Cytoskeleton and their underlying genotype is poorly understood. 22 To explore the genetiC meChanism of morphologiCal evolution of the eukaryotiC Cytoskeleton we 23 provide the first single Cell transCriptomes from unCultivable, free-living uniCellular eukaryotes: the 24 radiolarian speCies Lithomelissa setosa and Sticholonche zanclea. -
The Origin of Plastids By: Cheong Xin Chan, Ph.D
A Collaborative Learning Space for Science ABOUT FACULTY STUDENTS INTERMEDIATE CELL ORIGINS AND METABOLISM | Lead Editor: Gary Cote, Mario De Tullio The Origin of Plastids By: Cheong Xin Chan, Ph.D. & Debashish Bhattacharya, Ph.D. (Rutgers, The State University of New Jersey) © 2010 Nature Education Citation: Chan, C. X. & Bhattacharya, D. (2010) The Origin of Plastids. Nature Education 3(9):84 Plastids are core components of photosynthesis in plants and algae. Scientists are currently debating the events leading to the appearance of plastids in eukaryotic cells. Organelles, called plastids, are the main sites of photosynthesis in eukaryotic cells. Chloroplasts, as well as any other pigment containing cytoplasmic organelles that enables the harvesting and conversion of light and carbon dioxide into food and energy, are plastids. Found mainly in eukaryotic cells, plastids can be grouped into two distinctive types depending on their membrane structure: primary plastids and secondary plastids. Primary plastids are found in most algae and plants, and secondary, more-complex plastids are typically found in plankton, such as diatoms and dinoflagellates. Exploring the origin of plastids is an exciting field of research because it enhances our understanding of the basis of photosynthesis in green plants, our primary food source on planet Earth. Primary Plastids and Endosymbiosis Where did plastids originate? Their origin is explained by endosymbiosis, the act of a unicellular heterotrophic protist engulfing a free-living photosynthetic cyanobacterium and retaining it, instead of digesting it in the food vacuole (Margulis 1970; McFadden 2001; Kutschera & Niklas 2005). The captured cell (the endosymbiont) was then reduced to a functional organelle bound by two membranes, and was transmitted vertically to subsequent generations. -
Ocean Sciences Meeting 2020
Thursday: Ocean Sciences Meeting 2020 Session Information Moderators: Damien Josset, US Naval Research Laboratory; David Ortiz-Suslow, Naval Postgraduate Oral Sessions School; Helen Czerski, University College London Sessions are being held in the Convention Center (CC) Poster Sessions The eLightning Theater is located in Hall C-D (Poster Hall). 0800h AI41A-01 Meridional contrasts of the Southern Ocean Posters are on display in the following venue throughout the week: Hall C-D (Poster Hall) mixed layer in response to summer forcing: M du Plessis, S Swart, A F Thompson, P M S Monteiro, L C Biddle, S Session & Paper Numbering A Nicholson Paper Numbers - A paper number designates the section, or other 0815h AI41A-02 Laboratory measurements of ocean surface sponsoring group, and chronology of the presentation. drag in extreme wind and wave conditions: B K Haus, M Example: AI21A-01 = Air-Sea Interactions, Tuesday, AM, concurrent Curcic session AI, first paper in that session. 0830h AI41A-03 Noble Gas Fluxes Reveal Links Between Air- AI 2 1 A - 01 sea Gas Exchange, Bubbles, and the Structure of the Air- sea Interface at High Wind Speeds: R H Stanley, L Kinjo, A W Smith, H R Alt, C F N Krevanko, D Aldrett, Day Time E B Kopp, B K Haus 0845h AI41A-04 Wave-generated Turbulence in the Coastal 1 = Monday 1 = AM 0800–1000 Ocean During Passage of a Tropical Cyclone: A Gargett, 2 = Tuesday 2 = AM 1030–1230 D K Savidge 0900h AI41A-05 Modeling whitecaps on global scale: A 3 = Wednesday 3 = PM 1245–1345 / 1400–1600 Raman, A Darmenov 4 = Thursday 4 = PM 1600–1800 0915h AI41A-06 A two-layer model of whitecap spectral reectance: R J Frouin, B Fougnie, J Tan 5 = Friday 5 = PM 1830–2030 0930h AI41A-07 Spectral Energy Budget Analysis in the The program is current as of 04 February 2020. -
Systema Naturae. the Classification of Living Organisms
Systema Naturae. The classification of living organisms. c Alexey B. Shipunov v. 5.601 (June 26, 2007) Preface Most of researches agree that kingdom-level classification of living things needs the special rules and principles. Two approaches are possible: (a) tree- based, Hennigian approach will look for main dichotomies inside so-called “Tree of Life”; and (b) space-based, Linnaean approach will look for the key differences inside “Natural System” multidimensional “cloud”. Despite of clear advantages of tree-like approach (easy to develop rules and algorithms; trees are self-explaining), in many cases the space-based approach is still prefer- able, because it let us to summarize any kinds of taxonomically related da- ta and to compare different classifications quite easily. This approach also lead us to four-kingdom classification, but with different groups: Monera, Protista, Vegetabilia and Animalia, which represent different steps of in- creased complexity of living things, from simple prokaryotic cell to compound Nature Precedings : doi:10.1038/npre.2007.241.2 Posted 16 Aug 2007 eukaryotic cell and further to tissue/organ cell systems. The classification Only recent taxa. Viruses are not included. Abbreviations: incertae sedis (i.s.); pro parte (p.p.); sensu lato (s.l.); sedis mutabilis (sed.m.); sedis possi- bilis (sed.poss.); sensu stricto (s.str.); status mutabilis (stat.m.); quotes for “environmental” groups; asterisk for paraphyletic* taxa. 1 Regnum Monera Superphylum Archebacteria Phylum 1. Archebacteria Classis 1(1). Euryarcheota 1 2(2). Nanoarchaeota 3(3). Crenarchaeota 2 Superphylum Bacteria 3 Phylum 2. Firmicutes 4 Classis 1(4). Thermotogae sed.m. 2(5). -
The Maintenance and Replication of the Apicoplast Genome In
THE MAINTENANCE AND REPLICATION OF THE APICOPLAST GENOME IN TOXOPLASMA GONDII by SARAH BAKER REIFF (Under the Direction of Boris Striepen) ABSTRACT The phylum Apicomplexa comprises a group of intracellular parasites of significant global health and economic concern. Most apicomplexan parasites possess a relict plastid organelle, which no longer performs photosynthesis but still retains important metabolic functions. This organelle, known as the apicoplast, also contains its own genome which is required for parasite viability. The apicoplast and its genome have been shown to be useful as therapeutic targets. However, limited information is available about the replication and maintenance of plastid DNA, not just in apicomplexan parasites but also in plants and algae. Here we use the genetic tools available in the model apicomplexan Toxoplasma gondii to examine putative apicoplast DNA replication and condensation factors, including homologs of DNA polymerase I, single-stranded DNA binding protein, DNA gyrase, and the histone-like protein HU. We confirm targeting to the apicoplast of these candidates, which are all encoded in the nucleus and must be imported. We created a genetic knockout of the Toxoplasma HU gene, which encodes a homolog of a bacterial protein that helps condense the bacterial nucleoid. We show that loss of HU in Toxoplasma results in a strong decrease in apicoplast DNA content, accompanied by biogenesis and segregation defects of the organelle. We were also interested in examining the roles of enzymes that might be more directly involved in DNA replication. To this end we constructed conditional mutants of the Toxoplasma gyrase B homolog and the DNA polymerase I homolog, which appears to be the result of a gene fusion and contains multiple different catalytic domains. -
What Makes a Chloroplast? Reconstructing the Establishment of Photosynthetic Symbioses
Commentary 1865 What makes a chloroplast? Reconstructing the establishment of photosynthetic symbioses Richard G. Dorrell* and Christopher J. Howe Department of Biochemistry, University of Cambridge, Tennis Court Road, Cambridge CB2 1QW, UK *Author for correspondence ([email protected]) Journal of Cell Science 125, 1865–1875 ß 2012. Published by The Company of Biologists Ltd doi: 10.1242/jcs.102285 Summary Earth is populated by an extraordinary diversity of photosynthetic eukaryotes. Many eukaryotic lineages contain chloroplasts, obtained through the endosymbiosis of a wide range of photosynthetic prokaryotes or eukaryotes, and a wide variety of otherwise non- photosynthetic species form transient associations with photosynthetic symbionts. Chloroplast lineages are likely to be derived from pre- existing transient symbioses, but it is as yet poorly understood what steps are required for the establishment of permanent chloroplasts from photosynthetic symbionts. In the past decade, several species that contain relatively recently acquired chloroplasts, such as the rhizarian Paulinella chromatophora, and non-photosynthetic taxa that maintain photosynthetic symbionts, such as the sacoglossan sea slug Elysia, the ciliate Myrionecta rubra and the dinoflagellate Dinophysis, have emerged as potential model organisms in the study of chloroplast establishment. In this Commentary, we compare recent molecular insights into the maintenance of chloroplasts and photosynthetic symbionts from these lineages, and others that might represent the early stages of chloroplast establishment. We emphasise the importance in the establishment of chloroplasts of gene transfer events that minimise oxidative stress acting on the symbiont. We conclude by assessing whether chloroplast establishment is facilitated in some lineages by a mosaic of genes, derived from multiple symbiotic associations, encoded in the host nucleus. -
And Periplastid-Targeted Proteins in the Chlorarachniophyte Alga Bigelowiella Natans
GBE Proteomics Reveals Plastid- and Periplastid-Targeted Proteins in the Chlorarachniophyte Alga Bigelowiella natans Julia F. Hopkins1, David F. Spencer1, Sylvie Laboissiere2, Jonathan A.D. Neilson3,RobertJ.M.Eveleigh1, Dion G. Durnford3, Michael W. Gray1, and John M. Archibald1,* 1Department of Biochemistry and Molecular Biology, Dalhousie University, Nova Scotia, Canada 2Proteomics Unit, McGill University and Ge´nome Que´bec Innovation Centre, Quebec, Canada 3Department of Biology, University of New Brunswick, New Brunswick, Canada *Corresponding author: E-mail: [email protected]; [email protected]. Accepted: November 29, 2012 Abstract Chlorarachniophytes are unicellular marine algae with plastids (chloroplasts) of secondary endosymbiotic origin. Chlorarachniophyte cells retain the remnant nucleus (nucleomorph) and cytoplasm (periplastidial compartment, PPC) of the green algal endosymbiont from which their plastid was derived. To characterize the diversity of nucleus-encoded proteins targeted to the chlorarachniophyte plastid, nucleomorph, and PPC, we isolated plastid–nucleomorph complexes from the model chlorarachniophyte Bigelowiella natans and subjected them to high-pressure liquid chromatography-tandem mass spectrometry. Our proteomic analysis, the first of its kind for a nucleomorph-bearing alga, resulted in the identification of 324 proteins with 95% confidence. Approximately 50% of these proteins have predicted bipartite leader sequences at their amino termini. Nucleus-encoded proteins make up >90% of the proteins identified. With respect to biological function, plastid-localized light-harvesting proteins were well represented, as were proteins involved in chlorophyll biosynthesis. Phylogenetic analyses revealed that many, but by no means all, of the proteins identified in our proteomic screen are of apparent green algal ancestry, consistent with the inferred evolutionary origin of the plastid and nucleomorph in chlorarachniophytes.