Auroral Meridian Scanning Photometer Calibration Using Jupiter
Total Page:16
File Type:pdf, Size:1020Kb
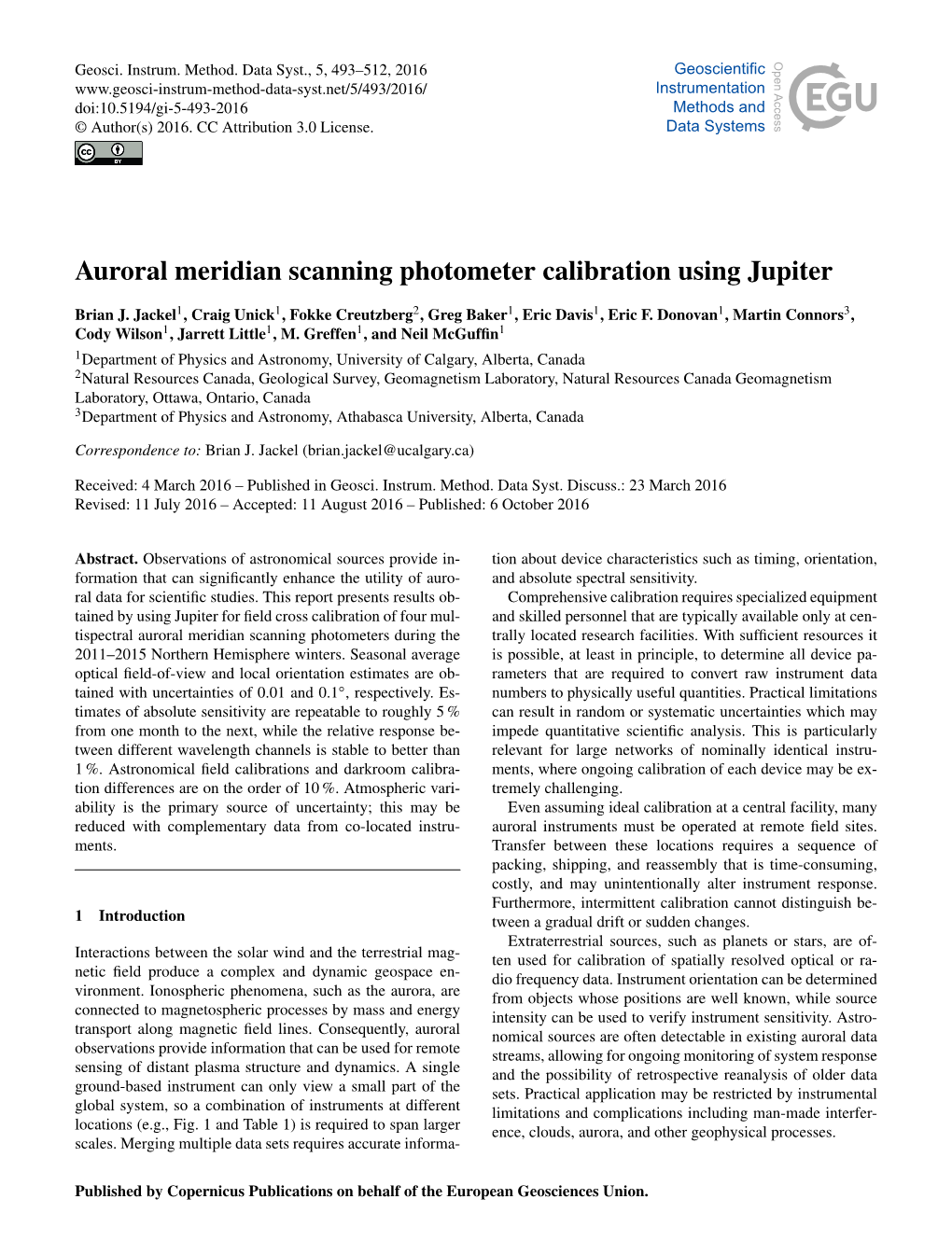
Load more
Recommended publications
-
Observational Astrophysics Lab Manual Lab 1
1 P139 - Observational Astrophysics Lab Manual Lab 1: Deriving the Mass of Jupiter or Saturn Professor T. Smecker-Hane Version 4.0 Sept 25, 2007 1 Introduction The mass of a planet can be determined by measuring the orbital period, τ, the time it takes to complete one orbit, and the orbital radius, A, a constant assuming the orbit is circular, of one of its moons. Doing this independently for a number of moons rather than just one allows you to decrease the error in your derived mass. For Jupiter, there are four easily observable moons. Listed in order from smallest to largest A are: Io, Europa, Ganymede and Callisto. These are commonly referred to as the Galilean moons, because Galileo discovered them in 1610. For Saturn, there are five easily observable moons. Listed in order from smallest to largest A are: Enceladus, Tethys, Dione, Rhea and Titan. 2 Theoretical Framework for the Experiment Use your knowledge of Newton’s laws and classical mechanics to determine the center of mass of the system composed of a planet of mass M and a moon of mass m. Recall that the two bodies both rotate around the center of mass with the period equal to τ. Assume that the moon’s orbit is circular, and the distance between the two in the orbital plane is r and r = A, where A is a constant for a circular orbit. Now determine τ as a function of A, m and M. Next take the limit of m << M, as is the case for all the moons of Jupiter and Saturn, and determine M as a function of A and τ. -
Translation to the Xephem Catalog Format
Translation of Some Star Catalogs to the XEphem Format Richard J. Mathar∗ Max-Planck Institute of Astronomy, K¨onigstuhl17, 69117 Heidelberg, Germany (Dated: August 28, 2020) The text lists Java programs which convert star catalogs to the format of the XEphem visualiser. The currently supported input catalog formats are (i) the data base of the orbital elements of the Minor Planet Center (MPC) of the International Astronomical Union (IAU), (ii) the data base of the Hipparcos main catalog or the variants of the Tycho-1 or Tycho-2 catalogs, (iii) the systems in the Washington Double Star catalog, (iv) the Proper Motions North and South catalogs, (v) the SKY2000 catalog, (vi) the 2MASS catalog, (vii) the Third Reference Catalog of Bright Galaxies (RC3), (viii) the General Catalogue of Variable Stars (GCVS v. 5). (ix) the Naval Observatory CCD Astrograph Catalog (UCAC4). [viXra:1802.0035] PACS numbers: 95.10.Km, 95.80.+p I. AIM AND SCOPE III. INVOCATION The Java library provided here supports loading star A. MPC Ephemerides catalogs into Downey's XEphem program to populate the chart of visible objects in sections of the sky, in the format Once the program and the auxiliary data files are com- of xephem 3.7.7. piled according to AppendixA, the program is run with The envisioned use is that the translated star catalogs a command line of the form can be included in the display by using the Data!Files gunzip MPCORB.DAT.gz menue of the xephem graphical user interface (GUI), and java -cp . de.mpg.mpia.xephem.Mpc2Xeph [ -a] by clicking on the Files menue of the GUI that pops up. -
A Satellite Constellation Visualization Program for Walkers and Lattice
BOUQUET: A SATELLITE CONSTELLATION VISUALIZATION PROGRAM FOR WALKERS AND LATTICE FLOWER CONSTELLATIONS A Thesis by MANDAKH ENKH Submitted to the Office of Graduate Studies of Texas A&M University in partial fulfillment of the requirements for the degree of MASTER OF SCIENCE August 2011 Major Subject: Aerospace Engineering Bouquet: A Satellite Constellation Visualization Program for Walkers and Lattice Flower Constellations Copyright 2011 Mandakh Enkh BOUQUET: A SATELLITE CONSTELLATION VISUALIZATION PROGRAM FOR WALKERS AND LATTICE FLOWER CONSTELLATIONS A Thesis by MANDAKH ENKH Submitted to the Office of Graduate Studies of Texas A&M University in partial fulfillment of the requirements for the degree of MASTER OF SCIENCE Approved by: Chair of Committee, Daniele Mortari Committee Members, John Hurtado John Junkins J. Maurice Rojas Head of Department, Dimitris Lagoudas August 2011 Major Subject: Aerospace Engineering iii ABSTRACT Bouquet: A Satellite Constellation Visualization Program for Walkers and Lattice Flower Constellations. (August 2011) Mandakh Enkh, B.S., Texas A&M University Chair of Advisory Committee: Dr. Daniele Mortari The development of the Flower Constellation theory offers an expanded framework to utilize constellations of satellites for tangible interests. To realize the full potential of this theory, the beta version of Bouquet was developed as a practical computer application that visualizes and edits Flower Constellations in a user-friendly manner. Programmed using C++ and OpenGL within the Qt software development environment for use on Windows systems, this initial version of Bouquet is capable of visualizing numerous user defined satellites in both 3D and 2D, and plot trajectories corresponding to arbitrary coordinate frames. The ultimate goal of Bouquet is to provide a viable open source alternative to commercial satellite orbit analysis programs. -
Skywatch Outbound!” Number 62 (New Series) 70S
Amateur Astronomy WITH AN ATTITUDE from Chaos Manor South! Rod Mollise’s May - June 2002 Volume 11, Issue 3 “A Newsletter for the Truly Skywatch Outbound!” Number 62 (New Series) 70s. There was this new scope <[email protected]> being advertised in Sky and Bustin’ Telescope. One that had hit amateur astronomy like a thunderbolt, the Inside this Issue: Schmidt Cassegrain in the form of the original Orange Tube C8. The Dew with new, mass-produced Celestron was the telescope that a lot of us had been waiting for. It combined Bustin’ Dew with the Dew elegant, useful features into a Buster! the Dew package that virtually made it a 1 portable observatory. Oh! how happy I was when I got my new Review of Xephem! Celestron out into the field for the 2 Buster! first time on a clear July evening. A “premium” dew heater I was happy for a while, anyway. 3 My Back Pages! controller… After a few hours I noticed that stars Rod Mollise weren’t as sharp as they had been. And the brighter ones were developing foggy little haloes. Was What do you do about dew? Do something wrong with my new you worry about dew? baby? Yep. A look at the corrector showed it to be a dripping mess. You do if you live in a location where Thus ended my first observing run high humidity makes nature’s little, with the Orange Tube. It was a nice damp gift a prime enemy of the demonstration of what happens working observer. Since I live on the when you point a big lens at the Gulf of Mexico coast, dew is an heat-sucking sky in a humid omnipresent fact of my observing environment: dew “falls” (or “forms,” life. -
Xephem Eks - I - ’Fem
XEphem eks - i - ’fem The Scientific-Grade Interactive Cross-Platform Astronomical Software Ephemeris UNIX − Mac OS X − MS Windows Version 3.5.2 User Manual Clear S ky I nstitute Copyright © 2002 Clear Sky Institute, Inc. All rights reserved. www.ClearSkyInstitute.com This document prepared on Linux kernel version 2.4.9-13 with Applixware version 4.4.2. Last changed January 14, 2002. Contents 1 XEphem -- the Interactive Ephemeris . 1-1 1.1 Summary of Capabilities. 1-1 1.2 UNIX Installation . 1-3 1.2.1 Launching XEphem . 1-5 1.3 Windows Installation . 1-6 1.3.1 Starting XEphem the First Time. 1-6 1.3.2 File Structure . 1-7 1.3.3 Multiple Users . 1-8 1.3.4 File naming . 1-9 1.3.5 Printing . 1-9 1.3.6 Uninstallation. 1-10 1.4 Apple Mac OS X Installation . 1-10 1.4.1 LX200 . 1-10 1.5 Operation . 1-10 1.6 Triad Formats. 1-11 2 Main Window . 2-1 2.1 File menu . 2-4 2.1.1 Network setup . 2-5 2.1.2 External Time/Loc . 2-5 2.2 View menu . 2-6 2.3 Tools menu . 2-6 2.4 Data menu . 2-6 2.5 Preferences menu . 2-7 2.5.1 Save . 2-9 2.5.2 Save Fonts . 2-11 2.5.3 Save Colors. 2-12 2.6 Sites dialog . 2-13 2.7 Examples . 2-14 2.7.1 Sky tonight. 2-14 2.7.2 Solar eclipse path . -
Xephem Eks - I - ’Fem
XEphem eks - i - ’fem The Scientific-Grade Interactive Cross-Platform Astronomical Software Ephemeris UNIX − Mac OS X − MS Windows Version 3.5.2 User Manual Clear S ky I nstitute Copyright © 2002 Clear Sky Institute, Inc. All rights reserved. www.ClearSkyInstitute.com This document prepared on Linux kernel version 2.4.9-13 with Applixware version 4.4.2. Last changed January 14, 2002. Contents 1 XEphem -- the Interactive Ephemeris . 1-1 1.1 Summary of Capabilities. 1-1 1.2 UNIX Installation . 1-3 1.2.1 Launching XEphem . 1-5 1.3 Windows Installation . 1-6 1.3.1 Starting XEphem the First Time. 1-6 1.3.2 File Structure . 1-7 1.3.3 Multiple Users . 1-8 1.3.4 File naming . 1-9 1.3.5 Printing . 1-9 1.3.6 Uninstallation. 1-10 1.4 Apple Mac OS X Installation . 1-10 1.4.1 LX200 . 1-10 1.5 Operation . 1-10 1.6 Triad Formats. 1-11 2 Main Window . 2-1 2.1 File menu . 2-4 2.1.1 Network setup . 2-5 2.1.2 External Time/Loc . 2-5 2.2 View menu . 2-6 2.3 Tools menu . 2-6 2.4 Data menu . 2-6 2.5 Preferences menu . 2-7 2.5.1 Save . 2-9 2.5.2 Save Fonts . 2-11 2.5.3 Save Colors. 2-12 2.6 Sites dialog . 2-13 2.7 Examples . 2-14 2.7.1 Sky tonight. 2-14 2.7.2 Solar eclipse path . -
IPSZILON Szeminárium, 2006 Szeptember 20
Szabad ég előtt Két planetáriumprogram: az XEphem és a KStars Holl András MTA Konkoly-Thege Miklós Csillagászati Kutatóintézet IPSZILON szeminárium, 2006 szeptember 20 - Az előadás célja - kikapcsolódás és művelődés; az égbolt: az elidegenített természet része - csillagászati ismeretterjesztés - a csillagászat, mint eszköz a földrajz, fizika, matematika és a számítástechnika oktatására - a csillagászat a szabadon hozzáférhető adatok, szolgáltatások, szoftverek, valamint az erős nemzetközi együttműködés miatt kiválóan alkalmas a kooperatív számítástechnikai alkalmazások bemutatására - Szabad planetáriumprogramok: XEphem, Stellarium, Celestia, KStars, Cartes du Ciel - programismertetők: Meteor (MCSE, http://meteor.mcse.hu) Celestia (GPL) http://celestia.sourceforge.net/ Linuxvilág #67 VII. évf. 8. sz. 2006 aug. 47. o. Meteor 2003/3 Stellarium (GPL) http://sourceforge.net/projects/stellarium/ http://stellarium.free.fr/ Meteor 2004/3 Mindkettő inkább a látványra helyezi a hangsúlyt Cartes du Ciel (GPL) http://www.ap-i.net/skychart/index.php Windows és Linux kettőt mutatunk be: - XEphem © 1990-2005 Elwood Charles Downey http://www.clearskyinstitute.com/ Linux reaches for the stars Linux Magazine, 2000, 10, 105 http://www.linux-magazine.com/issue/01/XEphem.pdf - KStars KDE - Edutainment Copyright © 2001, 2002, 2003 Jason Harris and the KStars Team http://edu.kde.org/kstars/index.php - Általános jellemzők - látvány - égbolt: csillagok, mély-ég objektumok (halmazok, galaxisok, ködök), csillagképek, tejút, koordináta-háló - naprendszer - a naprendszer égitestjei: Nap, Föld, Hold, bolygók (holdjaikkal) - kalkulátor, információk - külső adatforrások: - katalógusok (X) - aktuális képek: SOHO Nap (X); Föld meteo(X); - képek: DSS égbolt; HST (K); SEDS (K); stb. - információ: Wikipedia (K) - változócsillag fénygörbe (AAVSO) - seti@home támogatás (X) - saját adatok bevitele: FITS kép - WCS illesztés (X) - távcsővezérlés: soros v. -
Xephem Programismertető
Programismertető IPSZILON szeminárium Holl András, 2006 szeptember 20. XEphem C.E. Downey http://www.clearskyinstitute.com Tesztelt változat: Ver. 3.7.1 (2005 nov.), Fedora Linux, Sun Solaris 8 (továbbá 3.2.3, 3.4 Solaris 8) Általában minden Unix rendszeren futtatható (Windows alatt X-emulációval) pontos planetáriumprogram. Létezik ingyenes és megvásárolható verzió, a programkód hozzáférhető, de csupán portolás céljára módosítható. Az 1990-es évek eleje óta hozzáférhető, megtalálható volt professzionális és amatőr csillagászati szoftver CD-ken. Esetenként tudományos pontosságú, de nem minden vonatkozásban egyformán pontos. Nem tudományos alkalmazásokra pontossága kiváló, nagyobb pontosság-igény esetén már csillagászati ismereteket kívánhat. ----------------------------------------------------------------------- - Telepítés. A fejlesztő forráskódban teszi közre. Csupán a Motif könyvtárak helyét kell megadni - ha nincsenek, pl. lesstif használható. A neten található OpenMotif könyvtárral statikusan linkelt RPM csomag RedHat/Fedora Linuxhoz, illetve Source RPM. - Kezdeti beállítások. A számítógép óráját pontosan be kell állítani a helyi zónaidő szerint. Budapesti megfigyelési hely választása esetén korrigálni kell az xephem_sites állományban a bejegyzés hibás zónaidő-definícióját <xephemdir>/auxil/xephem_sites : -1 -re kell állítani a Greenwich-i időtől való eltérést (helyeset ld. Vienna). - Fő ablak: szükség esetén itt is beállíthatjuk az időt, helyszínt (lehet újat definiálni). Legtöbbször a tengerszint feletti magasság, hőmérséklet, légnyomás -
Anatomy of an Imaging System
Anatomy of an Imaging System What toys do you need on a Mac /Linux? Session 3 Anatomy of an Imaging System 3 separate sessions: 1. Description of the hardware and software generally 2. PC software integration and demo 3. Mac/Unix Raspberry Pi software integration and demo MAC and Linux software There are fewer software packages for OS X and Linux systems… • Planning & Observation: • Capturing & Processing: • Utilities: • AstroPlanner • ASTAP • CloudMakers FITS Preview • Observatory • Astro Pixel Processor • CloudMakers INDI Control Panel • Cartes du Ciel • Stark Labs Nebulosity • CloudMakers INDI Server • SkySafari 6 Pro • PixInsight • CloudMakers Astrometry • CloudMakers AstroImager • Collimation Aid • Planetarium & Scope • CloudMakers AstroDSLR • Darklight Control: • CloudMakers AstroGuider • SER Player • CloudMakers • PHD2 Guiding • Nightlight AstroTelescope • Open Astro Project • QFitsView • Stellarium • Lynkeos • PHD2 Log Viewer • KStars with EKOS • Keith's Image Stacker • Astroberry Server (RPi only) • StarTools • SkySafari 6 Pro • FireCapture • Starry Night • SiriL • TheSkyX • Planetary Imager • AstroGrav • ASTAP • CCDciel • StarStaX • Startrails Creator • AstroImageJ *https://www.macobservatory.com/mac-astronomy-software/ Also https://www.macobservatory.com/blog/2020/3/29/how-to-connect-your-telescope-to-your-home-network-with-kstarsekos MAC and Linux software We’ll focus on – Open-source KStars/Ekos (Windows / OS X / Linux) which integrates: ü Planetarium software ü Telescope / mount control drivers ü Camera control drivers ü -
2006 Melbourne
CONFERENCE PROCEEDINGS Sponsors The IPS2006 Conference Committee gratefully acknowledges the support of the following sponsors. Hypernova Sponsors Satchel Insert Sponsors Evans & Sutherland Learning Technologies Sky-Skan Inc. Lunar and Planetary Institute Supernova Sponsors National Space Centre American Museum of Natural History Swinburne University of Technology Carl Zeiss Note Pads Sponsor GOTO INC Evans & Sutherland RSA Cosmos Pens Sponsor SEOS Ltd. Carl Zeiss Spitz Inc. Conference Host Nova Sponsors Melbourne Planetarium, Scienceworks Audio Visual Imagineering Inc. Museum Victoria Barco Konica Minolta Planetarium Co. Ltd. How To…. The following page provides a table of contents for the proceedings. Each paper can be accessed from the table of contents by clicking on the title of the paper. As you enjoy reading through the papers, please take note that all figures are hyperlinked; simply click on the figure to follow the link and access full-sized figures. The authors for each paper can be contacted through their hyperlinked email addresses. Many thanks to all who have contributed to the proceedings. Proceedings Editor Dr Tanya Hill Curator, Astronomy Melbourne Planetarium, Scienceworks Museum Victoria 2 Booker St, Spotswood 3105 Victoria Australia Phone: +61 3 9392 4503 Fax: +61 3 9391 0100 Email: [email protected] Table of Contents – Author Index A Permanent Planetarium For Sydney M. W. B. Anderson, G. Nicholson, S. Fleming and A. Scarvell Masters Students In Science Communication Are Educated For Work At Planetariums Lars Broman What A Difference Evaluation Makes Martin Bush and Carolyn Meehan Native Brazilian Skies Alexandre Cherman Puppets in the Dome Alexandre Cherman Aboriginal Skies Paul Curnow From Analog To Digital: A Case Study Dale A. -
Xephem Reference Manual
XEphem file:///home/ecdowney/telescope/GUI/xephem/help/xephem.html XEphem pronounced eks i fem´ Version 3.7.3 Reference Manual © 1990-2008 Elwood Charles Downey 1. Introduction 1. Quantitative information 2. Local circumstances 3. Launching XEphem 1. Shared and Private Directories 2. Main window control 4. Time and angle formats 2. Main Window 1. Main's Help menu 2. Menu bar 1. File 2. View 3. Tools 4. Data 5. Preferences 3. Sections 1. Local 1. Site Selection 2. Time 3. Calendar 4. Night 5. Looping 3. File menu 1. System log 2. Gallery 1. File format 3. Network Setup 4. External Input 4. View menu 1. Data Table 1. Data setup 2. Sun 1. Sun mouse 2. Sun Control menu 3. Sun Files menu 4. Sun Type menu 5. Sun Size menu 1 of 106 03/21/08 16:40 XEphem file:///home/ecdowney/telescope/GUI/xephem/help/xephem.html 3. Moon 1. Moon mouse 2. Moon Control menu 3. Moon View menu 1. More info... 4. Scale menu 5. Lunar Orbiter IV 4. Earth 1. Earth mouse 2. Earth Control menu 3. Earth View menu 1. Objects dialog 5. Mars 1. Mars mouse 2. Mars Control menu 3. Mars View menu 1. Features... 2. More info... 3. Moon view... 6. Jupiter 1. Jupiter mouse 2. Jupiter Control menu 3. Jupiter View menu 1. More info... 7. Saturn 1. Saturn mouse 2. Saturn Control menu 3. Saturn View menu 1. More info... 8. Uranus 1. Uranus mouse 2. Uranus Control menu 3. Uranus View menu 1. More info.. -
Studies of the Influence of Moonlight on Observations with the MAGIC
Studies of the Influence of Moonlight on Observations with the MAGIC Telescope Daniel Britzger Munchen¨ 2009 Studies of the Influence of Moonlight on Observations with the MAGIC Telescope Daniel Britzger Diplomarbeit an der Fakult¨at fur¨ Physik der Ludwig{Maximilians{Universit¨at Munchen¨ vorgelegt von Daniel Britzger aus Marktoberdorf Munchen,¨ den 30. Juni 2009 Erstgutachter: Prof. Dr. C. Kiesling Zweitgutachter: Prof. Dr. D. Schaile Zusammenfassung Die hier vorgestellte Diplomarbeit behandelt ein Thema auf dem Gebiet der ergebunde- nen Gammastrahlenastronomie mit grossen Cherenkovteleskopen. Es geht dabei speziell um die Erweiterung der Messzeit durch Beobachtungen bei schwachem Mondlicht. Die ergebundene Gammastrahlenastronomie ist ein sehr erfolgreiches Teilgebiet der Hoch- energie Astroteilchenphysik, einem neuen und rasch expandierenden Bereich der Grund- lagenforschung. Nahzeu alle Resultate in der ergebundenen Gammastrahlen Astronomie wurden mit Cherenkovteleskopen erzielt. Mit diesen Teleskopen beobachtet man bei klaren, dunklen N¨achten das Cherenkovlicht das von Luftschauern in der Atmosph¨are abgestrahlt wird. Diese Luftschauer werden durch kosmische Teilchen, u.a. hochenerge- tische, kosmische Gammaphotonen beim Auftreffen auf die Atmosph¨are erzeugt. Eines der erfolgreichsten Teleskope auf diesem Gebiet ist das MAGIC Teleskop. Das MAGIC-I Teleskop auf der Kanareninsel La Palma hat eine parabolische Spiegelfl¨ache von 234 m2 und ist zur Zeit das weltgr¨oßte Teleskop mit der niedrigsten Energieschwelle. Die Ana- lyse von sehr schwachen und nur einige Nanosekunden (∼ 3 ns) dauernden Lichtblitzen, verursacht durch die Cherenkovstrahlung von Sekund¨arteilchen in einem ausgedehntem Luftschauer, erlaubt die Rekonstruktion der elektromagnetischen, bzw. hadronischen Kaskaden. So ist eine Klassifikation des Prim¨arteilchens, seiner Energie sowie der An- kunftsrichtung m¨oglich. Im Falle von Gammaquanten erlaubt dies auch die Zuordnung zu stellaren Objekten im Weltall.