Satellite Altimetry and Key Observations: What We've Learned, and Learned, We've What Altimetry Andkey Observations: Satellite - Lueng Fu
Total Page:16
File Type:pdf, Size:1020Kb
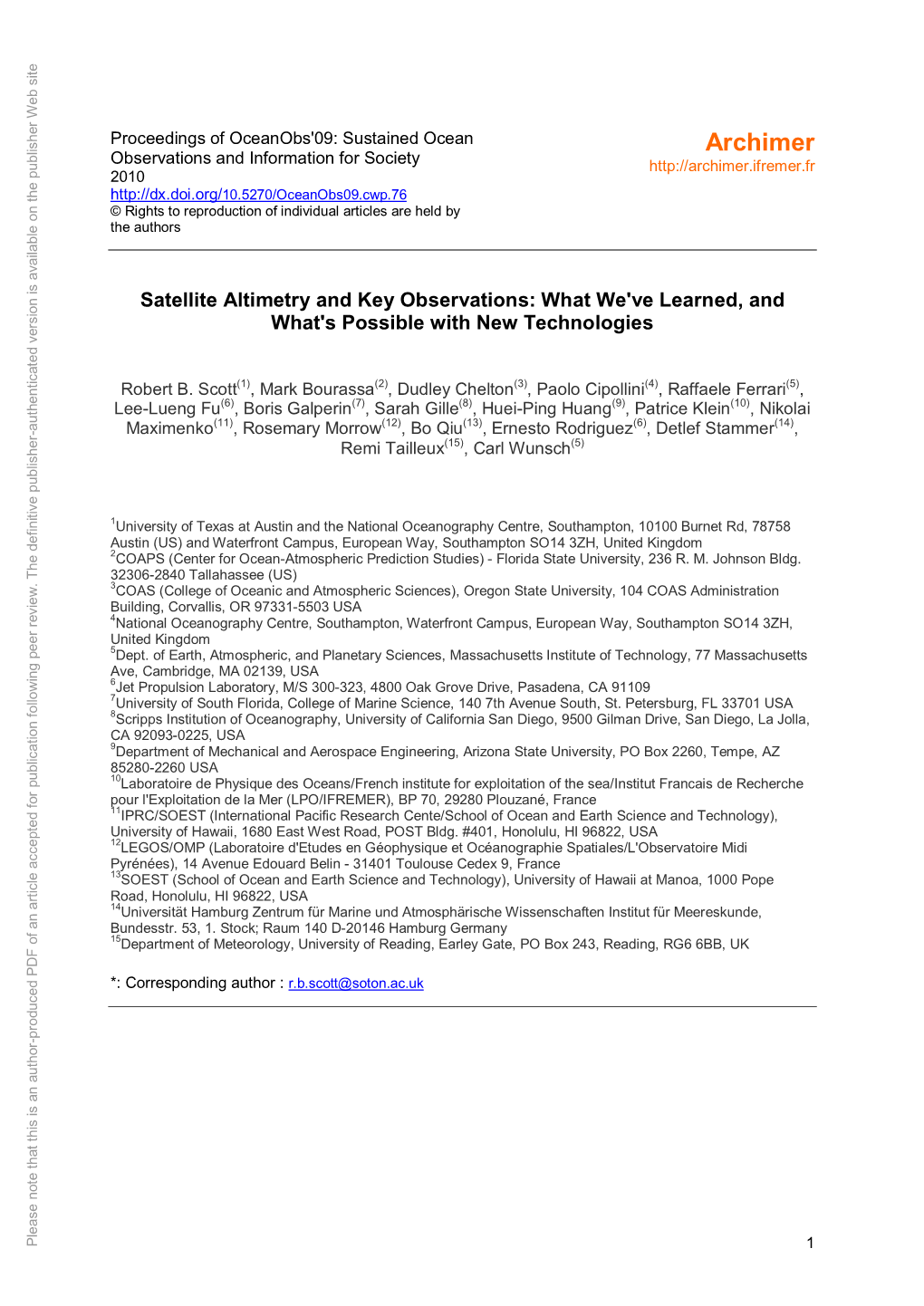
Load more
Recommended publications
-
Ocean Surface Topography Altimetry by Large Baseline Cross-Interferometry from Satellite Formation
remote sensing Article Ocean Surface Topography Altimetry by Large Baseline Cross-Interferometry from Satellite Formation Weiya Kong 1,2, Bo Liu 2,*, Xiaohong Sui 2, Running Zhang 3 and Jinping Sun 1 1 School of Electronic and Information Engineering, Beihang University, Beijing 100191, China; [email protected] (W.K.); [email protected] (J.S.) 2 Qian Xuesen Laboratory of Space and Technology, Beijing 100094, China; [email protected] 3 Beijing Institute of Spacecraft System Engineering, Beijing 100094, China; [email protected] * Correspondence: [email protected]; Tel.: +86-010-6811-3401 Received: 21 September 2020; Accepted: 26 October 2020; Published: 27 October 2020 Abstract: Imaging Radar Altimeter (IRA) is the current development tendency for ocean surface topography (OST) altimetry,which utilizes Synthetic Aperture Radar (SAR) and interferometry to improve the spatial resolution of OST to several kilometers or even better. Meanwhile, centimetric altimetry accuracy should be guaranteed for applications such as geostrophic currents or marine gravity anomaly inversion. However, the baseline length of IRA which determines the altimetric sensitivity is confined by the satellite platform, in consideration of baseline vibration and payload capability. Therefore, the baseline length from a single satellite can extend to only tens of meters, making it difficult to achieve centimetric accuracy. Referring to the successful experience from TerraSAR-X/TanDEM-X, satellite formation can easily extend the baseline length to hundreds or thousands of meters, depending on the helix orbit. Therefore, we propose the large baseline IRA (LB-IRA) from satellite formation for OST altimetry: the carrier frequency shift (CFS) is brought in to compensate for the severe baseline decorrelation, and the helix orbit is carefully selected to prevent severe time decorrelation from along-track baseline. -
Global Observations of Fine-Scale Ocean Surface Topography with the Surface Water and Ocean Topography (SWOT) Mission
fmars-06-00232 May 13, 2019 Time: 15:5 # 1 REVIEW published: 15 May 2019 doi: 10.3389/fmars.2019.00232 Global Observations of Fine-Scale Ocean Surface Topography With the Surface Water and Ocean Topography (SWOT) Mission Rosemary Morrow1*, Lee-Lueng Fu2, Fabrice Ardhuin3, Mounir Benkiran4, Bertrand Chapron3, Emmanuel Cosme5, Francesco d’Ovidio6, J. Thomas Farrar7, Sarah T. Gille8, Guillaume Lapeyre9, Pierre-Yves Le Traon4, Ananda Pascual10, Aurélien Ponte3, Bo Qiu11, Nicolas Rascle12, Clement Ubelmann13, Jinbo Wang2 and Edward D. Zaron14 1 Centre de Topographie des Océans et de l’Hydrosphère, Laboratoire d’Etudes en Géophysique et Océanographie Spatiale, CNRS, CNES, IRD, Université Toulouse III, Toulouse, France, 2 Jet Propulsion Laboratory, California Institute of Technology, Pasadena, CA, United States, 3 Laboratoire d’Océanographie Physique et Spatiale, Centre National de la Edited by: Recherche Scientifique – Ifremer, Plouzané, France, 4 Mercator Ocean, Ramonville-Saint-Agne, France, 5 Institut des Fei Chai, Géosciences de l’Environnement, Université Grenoble Alpes, Grenoble, France, 6 Sorbonne Université, CNRS, IRD, MNHN, Second Institute of Oceanography, Laboratoire d’Océanographie et du Climat: Expérimentations et Approches Numériques (LOCEAN-IPSL), Paris, France, State Oceanic Administration, China 7 Woods Hole Oceanographic Institution, Woods Hole, MA, United States, 8 Scripps Institution of Oceanography, University 9 Reviewed by: of California, San Diego, La Jolla, CA, United States, Laboratoire de Météorologie Dynamique (LMD-IPSL), -
Climate-Change–Driven Accelerated Sea-Level Rise Detected in the Altimeter Era
Climate-change–driven accelerated sea-level rise detected in the altimeter era R. S. Nerema,1, B. D. Beckleyb, J. T. Fasulloc, B. D. Hamlingtond, D. Mastersa, and G. T. Mitchume aColorado Center for Astrodynamics Research, Ann and H. J. Smead Aerospace Engineering Sciences, Cooperative Institute for Research in Environmental Sciences, University of Colorado, Boulder, CO 80309; bStinger Ghaffarian Technologies Inc., NASA Goddard Space Flight Center, Greenbelt, MD 20771; cNational Center for Atmospheric Research, Boulder, CO 80305; dOld Dominion University, Norfolk, VA 23529; and eCollege of Marine Science, University of South Florida, St. Petersburg, FL 33701 Edited by Anny Cazenave, Centre National d’Etudes Spatiales, Toulouse, France, and approved January 9, 2018 (received for review October 2, 2017) Using a 25-y time series of precision satellite altimeter data from acceleration estimate by 0.033 mm/y2, resulting in a final “climate- TOPEX/Poseidon, Jason-1, Jason-2, and Jason-3, we estimate the change–driven” acceleration of 0.084 mm/y2. Climate-change–driven climate-change–driven acceleration of global mean sea level over in this case means we have tried to adjust the GMSL measurements the last 25 y to be 0.084 ± 0.025 mm/y2. Coupled with the average for as many natural interannual and decadal effects as we can to try climate-change–driven rate of sea level rise over these same 25 y of to isolate the longer-term, potentially anthropogenic, acceleration–– 2.9 mm/y, simple extrapolation of the quadratic implies global mean any remaining effects are considered in the error analysis. sea level could rise 65 ± 12 cm by 2100 compared with 2005, roughly We also must consider the impact of errors in the altimeter in agreement with the Intergovernmental Panel on Climate Change measurements, especially instrument drift. -
2019 Ocean Surface Topography Science Team Meeting Convene
2019 Ocean Surface Topography Science Team Meeting Convene Chicago 16 West Adams Street, Chicago, IL 60603 Monday, October 21 2019 - Friday, October 25 2019 The 2019 Ocean Surface Topography Meeting will occur 21-25 October 2019 and will include a variety of science and technical splinters. These will include a special splinter on the Future of Altimetry (chaired by the Project Scientists), a splinter on Coastal Altimetry, and a splinter on the recently launched CFOSAT. In anticipation of the launch of Jason-CS/Sentinel-6A approximately 1 year after this meeting, abstracts that support this upcoming mission are highly encouraged. Abstracts Book 1 / 259 Abstract list 2 / 259 Keynote/invited OSTST Opening Plenary Session Mon, Oct 21 2019, 09:00 - 12:35 - The Forum 12:00 - 12:20: How accurate is accurate enough?: Benoit Meyssignac 12:20 - 12:35: Engaging the Public in Addressing Climate Change: Patricia Ward Science Keynotes Session Mon, Oct 21 2019, 14:00 - 15:45 - The Forum 14:00 - 14:25: Does the large-scale ocean circulation drive coastal sea level changes in the North Atlantic?: Denis Volkov et al. 14:25 - 14:50: Marine heat waves in eastern boundary upwelling systems: the roles of oceanic advection, wind, and air-sea heat fluxes in the Benguela system, and contrasts to other systems: Melanie R. Fewings et al. 14:50 - 15:15: Surface Films: Is it possible to detect them using Ku/C band sigmaO relationship: Jean Tournadre et al. 15:15 - 15:40: Sea Level Anomaly from a multi-altimeter combination in the ice covered Southern Ocean: Matthis Auger et al. -
The Difference of Sea Level Variability by Steric Height and Altimetry In
remote sensing Letter The Difference of Sea Level Variability by Steric Height and Altimetry in the North Pacific Qianran Zhang 1, Fangjie Yu 1,2,* and Ge Chen 1,2 1 College of Information Science and Engineering, Ocean University of China, Qingdao 266100, China; [email protected] (Q.Z.); [email protected] (G.C.) 2 Laboratory for Regional Oceanography and Numerical Modeling, Qingdao National Laboratory for Marine Science and Technology, Qingdao 266200, China * Correspondence: [email protected]; Tel.: +86-0532-66782155 Received: 4 December 2019; Accepted: 22 January 2020; Published: 24 January 2020 Abstract: Sea level variability, which is less than ~100 km in scale, is important in upper-ocean circulation dynamics and is difficult to observe by existing altimetry observations; thus, interferometric altimetry, which effectively provides high-resolution observations over two swaths, was developed. However, validating the sea level variability in two dimensions is a difficult task. In theory, using the steric method to validate height variability in different pixels is feasible and has already been proven by modelled and altimetry gridded data. In this paper, we use Argo data around a typical mesoscale eddy and altimetry along-track data in the North Pacific to analyze the relationship between steric data and along-track data (SD-AD) at two points, which indicates the feasibility of the steric method. We also analyzed the result of SD-AD by the relationship of the distance of the Argo and the satellite in Point 1 (P1) and Point 2 (P2), the relationship of two Argo positions, the relationship of the distance between Argo positions and the eddy center and the relationship of the wind. -
Lesson Plans Graphing Sea Level Slopes and Surface Currents
My NASA Data - Lesson Plans Graphing Sea Level Slopes and Surface Currents Purpose Students analyze the relationship between sea surface height and ocean surface currents by graphing sea height using satellite data. Note: This lesson is modified from NASA's TOPEX/Poseidon lesson plan. Learning Objectives Describe the use of a radar altimeter to measure sea surface height. Plot sea surface height data. Describe the relationship between the slope of the sea surface and the direction and speed of ocean surface currents. Why Does NASA Study This Phenomenon? The ocean surface is not level but has broad, gradual hills and valleys created by surface winds and density differences. Surface currents flow around the sides of these hills and valleys. Measuring this sea surface topography is a challenging task. One measuring device is the TOPEX/Poseidon radar altimeter mounted on an Earth-orbiting satellite. This device sends radar beams down to the sea surface, where they are reflected back to the satellite. The round-trip travel times for the beams allow 1 / 7 scientists to measure the satellite to sea surface distance to within a few centimeters. The satellite- derived sea surface elevations are then compared to those that the sea surface would have if the oceans were still (no currents, waves, etc.). Specifically, the elevations of the imaginary still ocean are subtracted from those calculated from the satellite's data. The height differences show where the ocean's hills and valleys are and the slope of the surface between them. The following activity uses some of these sea height differences, calculated from TOPEX/Poseidon data to investigate the relationship between sea surface topography and ocean surface currents. -
Comparison of Two Methods to Assess Ocean Tide Models
AUGUST 2012 W A N G E T A L . 1159 Comparison of Two Methods to Assess Ocean Tide Models XIAOCHUN WANG JIFRESSE, University of California, Los Angeles, Los Angeles, California YI CHAO Jet Propulsion Laboratory, California Institute of Technology, Pasadena, California C. K. SHUM,YUCHAN YI, AND HOK SUM FOK Division of Geodetic Science, School of Earth Sciences, Ohio State University, Columbus, Ohio (Manuscript received 13 September 2011, in final form 10 April 2012) ABSTRACT Two methods to assess ocean tide models, the current method and the total discrepancy method, are com- pared from the perspective of their relationship to the root-mean-square difference of tidal sea surface height (total discrepancy). These two methods are identically the same when there is only one spatial location involved. When there is more than one spatial location involved, the current method is the root-mean-square difference of total discrepancy at each location, and the total discrepancy method is the averaged total discrepancy. The result from the current method is always larger than or equal to that from the total discrepancy method. Monte Carlo simulation indicates that the difference between their results increases with increasing spatial variability of total discrepancy. Both of these two methods are then used to compare the two tide models of the Ocean Surface Topography Mission (OSTM)/Jason-2. The discrepancy of these two models as measured by the total discrepancy method decreases monotonically from around 11.4 to 2.2 cm with depth increasing from 50 to 1000 m. In contrast, the discrepancy measured by the current method varies from 21.6 to 2.9 cm. -
Ocean Surface Topography Mission/ Jason 2 Launch
PREss KIT/JUNE 2008 Ocean Surface Topography Mission/ Jason 2 Launch Media Contacts Steve Cole Policy/Program Management 202-358-0918 Headquarters [email protected] Washington Alan Buis OSTM/Jason 2 Mission 818-354-0474 Jet Propulsion Laboratory [email protected] Pasadena, Calif. John Leslie NOAA Role 301-713-2087, x174 National Oceanic and [email protected] Atmospheric Administration Silver Spring, Md. Eliane Moreaux CNES Role 011-33-5-61-27-33-44 Centre National d’Etudes Spatiales [email protected] Toulouse, France Claudia Ritsert-Clark EUMETSAT Role 011-49-6151-807-609 European Organisation for the [email protected] Exploitation of Meteorological Satellites Darmstadt, Germany George Diller Launch Operations 321-867-2468 Kennedy Space Center, Fla. [email protected] Contents Media Services Information ...................................................................................................... 5 Quick Facts .............................................................................................................................. 7 Why Study Ocean Surface Topography? ..................................................................................8 Mission Overview ....................................................................................................................13 Science and Engineering Objectives ....................................................................................... 20 Spacecraft .............................................................................................................................22 -
SWOT 101: a Mission Primer
SWOT Surface Water and Ocean Topography Mission – SWOT 101 A quantum improvement for oceanography and hydrology from the next generation altimeter mission Contributions by JPL, SSC, CNES, the SWOT Project, and the SWOT Applications teams More info: swot.jpl.nasa.gov http://www.aviso.altimetry.fr/swot Jet Propulsion Laboratory, California Institute of Technology. Copyright © 2015. All rights reserved. Jet Propulsion Laboratory, California Institute of Technology. Copyright © 2015. All rights reserved. 1 SWOT Outline • SWOT mission description • Oceanography • Hydrology • Synergistic science • Data products • Summary Jet Propulsion Laboratory, California Institute of Technology. Copyright © 2015. All rights reserved. 2 SWOT SWOT Mission Overview • The Surface Water and Ocean Topography mission (SWOT) is a satellite mission recommended by the US National Research Council 2007 Decadal Survey (DS) that will provide a quantum improvement for oceanography and hydrology • Oceanography: First global determination of the ocean circulation, kinetic energy and dissipation at high resolution • Hydrology: First global inventory of fresh water storage and its change on a global basis • Planned launch: September 2021 Jet Propulsion Laboratory, California Institute of Technology. Copyright © 2015. All rights reserved. 3 SWOT Major Partnership: NASA and CNES • Planned as a major partnership between NASA and the French Space Agency (CNES). • Continues a 25+ year partnership NASA and CNES of the highly successful altimetric oceanographic satellite missions -
Highways in the Coastal Environment: Assessing Extreme Events
Archival Superseded by HEC-25 3rd edition - January 2020 Publication No. FHWA-NHI-14-006 October 2014 U.S. Department of Hydraulic Engineering Circular No. 25 – Volume 2 Transportation Federal Highway Administration Archival Superseded by HEC-25 3rd edition - January 2020 Highways in the Coastal Environment: Assessing Extreme Events Archival Superseded by HEC-25 3rd edition - January 2020 TECHNICAL REPORT DOCUMENTATION PAGE Archival Superseded by HEC-25 3rd edition - January 2020 Form DOT F 1700.7 (8-72) Reproduction of completed page authorized Archival Superseded by HEC-25 3rd edition - January 2020 Table of Contents Table of Contents ........................................................................................................... i List of Figures ................................................................................................................ v List of Tables ................................................................................................................ vii Acknowledgements ........................................................................................................ ix Glossary ........................................................................................................................ xi List of Acronyms .......................................................................................................... xxi Chapter 1 – Introduction ................................................................................................ 1 1.1 Background ........................................................................................................ -
Ocean Surface Topography/Jason-2
National Aeronautics and Space Administration Ocean Surface Topography Mission/Jason-2 science writers’ guide May 2008 OSTM/Jason-2 SCIENCE WRITERS’ GUIDE CONTACT INFORMATION & MEDIA RESOURCES Please call the Public Affairs Offices at NASA, NOAA, CNES or EUMETSAT before contacting individual scientists at these organizations. NASA Jet Propulsion Laboratory Alan Buis, 818-354-0474, [email protected] NASA Headquarters Steve Cole, 202-358-0918, [email protected] NASA Kennedy Space Center George Diller, 321-867-2468, [email protected] NOAA Environmental Satellite, Data and Information Service John Leslie, 301-713-2087, x174, [email protected] CNES Eliane Moreaux, 011-33-5-61-27-33-44, [email protected] EUMETSAT Claudia Ritsert-Clark, 011-49-6151-807-609, [email protected] NASA Web sites http://www.nasa.gov/ostm http://sealevel.jpl.nasa.gov/mission/ostm.html NOAA Web site http://www.osd.noaa.gov/ostm/index.htm CNES Web site http://www.aviso.oceanobs.com/en/missions/future-missions/jason-2/index.html EUMETSAT Web site http://www.eumetsat.int/Home/Main/What_We_Do/Satellites/Jason/index.htm?l=en WRITERS Alan Buis Kathryn Hansen Gretchen Cook-Anderson Rosemary Sullivant OSTM/Jason-2 SCIENCE WRITERS’ GUIDE TABLE OF CONTENTS Science Overview............................................................................ 2 Instruments.................................................................................... 3 Feature Stories New Mission Helps Offshore Industries Dodge Swirling Waters ................................................................. -
OSTM/Jason–2 Extending the Time Series — Ocean Data from Space
National Aeronautics and Space Administration OSTM/Jason–2 Extending the Time Series — Ocean Data from Space http://sealevel.jpl.nasa.gov The Ocean Surface Topography Mission (OSTM) is the next-generation ocean al timetry mission to extend the time series of sea surface topography measurements begun by TOPEX/Poseidon (1992–2005) and continued by Jason-1 (2001– ). While the TOPEX/Poseidon and Jason-1 missions were collaborations between NASA and the French space agency CNES, OSTM is a four-partner mission with NASA, CNES, the European Organisation for the Exploitation of Meteorological Satellites (Eumetsat), and NOAA. The OSTM satellite (Jason-2), will carry the next genera tion instruments including CNES’s Poseidon-3 dual-frequency radar altimeter to measure sea surface height and NASA/JPL’s Advanced Microwave Radiometer (AMR) to remove the effects of water vapor from the altimetry measurement. With these and other technological improvements, OSTM will maintain or surpass Ja son-1’s measurement accuracy of 3.3 centimeters. This precise measurement will CNES/Mira Productions help scientists better understand ocean circulation and its effect on global climate. In combination with several other JPL-managed missions, including the Gravity Recovery and Climate Experiment, which measures Earth’s mass distribution, Advanced Microwave QuikScat, which measures near-surface ocean winds, and Aquarius (to be Radiometer launched in 2009) which measures ocean surface salinity, OSTM will make an Global Positioning (AMR) System Payload important contribution