Replication Stress: Mechanisms and Molecules Involved in DNA Replication Progression and Reinitiation
Total Page:16
File Type:pdf, Size:1020Kb
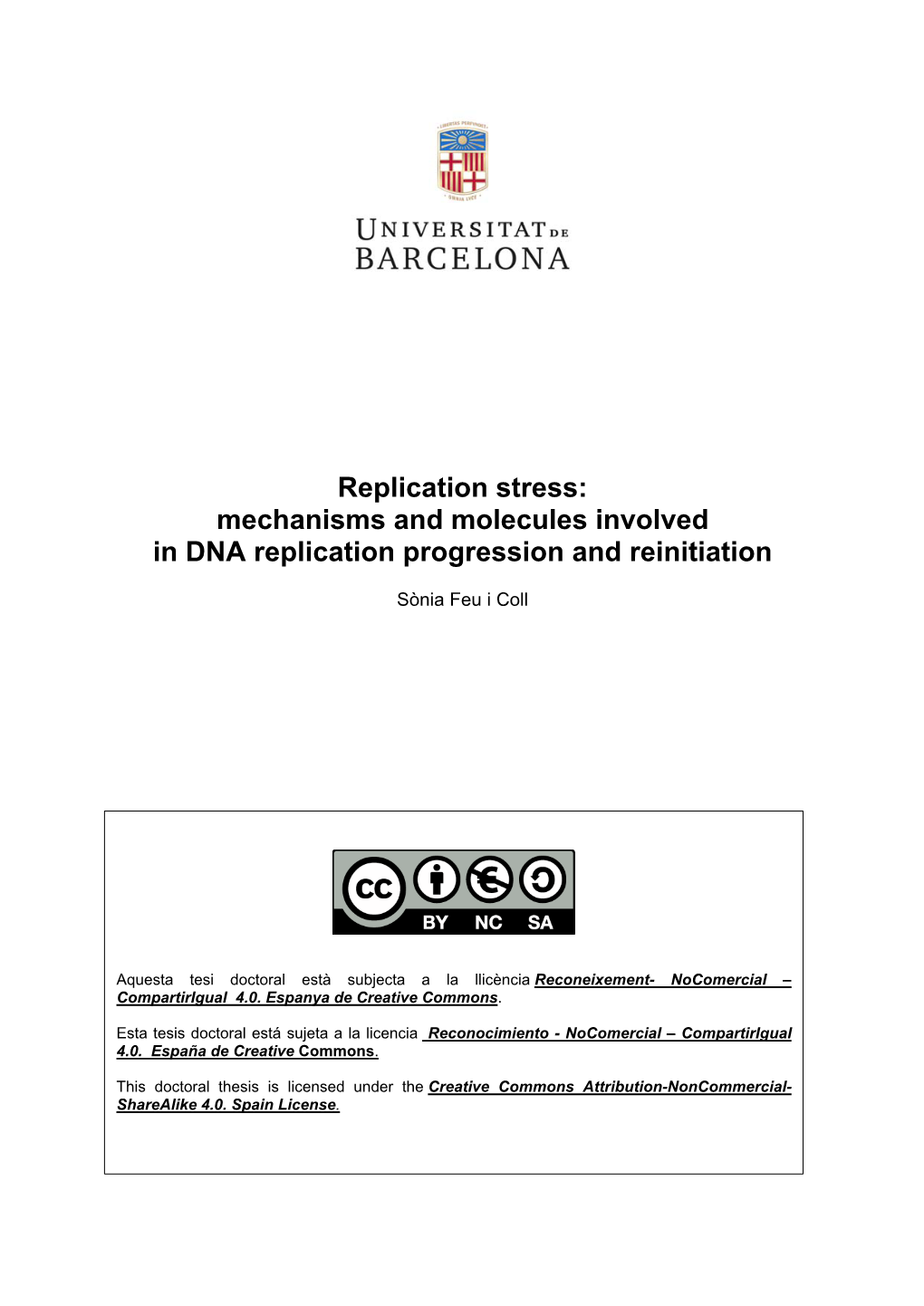
Load more
Recommended publications
-
Molecular Anatomy and Regulation of a Stable Replisome at a Paused Eukaryotic DNA Replication Fork
Downloaded from genesdev.cshlp.org on September 29, 2021 - Published by Cold Spring Harbor Laboratory Press Molecular anatomy and regulation of a stable replisome at a paused eukaryotic DNA replication fork Arturo Calzada,1,2,3 Ben Hodgson,1,3 Masato Kanemaki,1 Avelino Bueno,2 and Karim Labib1,4 1Paterson Institute for Cancer Research, Christie Hospital NHS Trust, Manchester M20 4BX, United Kingdom; 2Cancer Research Institute, University of Salamanca/CSIC, 37007 Salamanca, Spain Eukaryotic cells regulate the progression and integrity of DNA replication forks to maintain genomic stability and couple DNA synthesis to other processes. The budding yeast proteins Mrc1 and Tof1 associate with the putative MCM–Cdc45 helicase and limit progression of the replisome when nucleotides are depleted, and the checkpoint kinases Mec1 and Rad53 stabilize such stalled forks and prevent disassembly of the replisome. Forks also pause transiently during unperturbed chromosome replication, at sites where nonnucleosomal proteins bind DNA tightly. We describe a method for inducing prolonged pausing of forks at protein barriers assembled at unique sites on a yeast chromosome, allowing us to examine for the first time the effects of pausing upon replisome integrity. We show that paused forks maintain an intact replisome that contains Mrc1, Tof1, MCM–Cdc45, GINS, and DNA polymerases ␣ and and that recruits the Rrm3 helicase. Surprisingly, pausing does not require Mrc1, although Tof1 and Csm3 are both important. In addition, the integrity of the paused forks does not require Mec1, Rad53, or recombination. We also show that paused forks at analogous barriers in the rDNA are regulated similarly. These data indicate that paused and stalled eukaryotic replisomes resemble each other but are regulated differently. -
Incision of Damaged DNA in the Presence of an Impaired Smc5/6 Complex Imperils Genome Stability Jie Peng and Wenyi Feng*
10216–10229 Nucleic Acids Research, 2016, Vol. 44, No. 21 Published online 17 August 2016 doi: 10.1093/nar/gkw720 Incision of damaged DNA in the presence of an impaired Smc5/6 complex imperils genome stability Jie Peng and Wenyi Feng* Department of Biochemistry and Molecular Biology, State University of New York Upstate Medical University, Syracuse, NY 13210, USA Received February 01, 2016; Revised August 05, 2016; Accepted August 08, 2016 ABSTRACT cleotide excision repair (RER) pathways, respectively (7). / Virtually all DNA repair pathways require recognition and The Smc5 6 complex is implicated in homologous subsequent excisions of the damaged nucleotide, giving rise recombination-mediated DNA repair during DNA to a single-stranded gap in the DNA polymer (7). The mech- damage or replication stress. Here, we analysed anisms ensuring such a structural alteration of the chro- genome-wide replication dynamics in a hypomorphic mosome is efficiently repaired and does not lead to further budding yeast mutant, smc6-P4. The overall replica- degradation are underexplored. Conceivably, these mecha- tion dynamics in the smc6 mutant is similar to that nisms would involve protein complexes directly interacting in the wild-type cells. However, we captured a dif- with the chromosomal DNA and implicated in DNA dam- ference in the replication profile of an early S phase age or replication stress response. sample in the mutant, prompting the hypothesis that One of the three evolutionarily conserved Structure the mutant incorporates ribonucleotides and/or ac- Maintenance of Chromosome (SMC) protein complexes, the Smc5/6 complex, plays a key role in DNA damage re- cumulates single-stranded DNA gaps during replica- pair. -
Working on Genomic Stability: from the S-Phase to Mitosis
G C A T T A C G G C A T genes Review Working on Genomic Stability: From the S-Phase to Mitosis Sara Ovejero 1,2,3,* , Avelino Bueno 1,4 and María P. Sacristán 1,4,* 1 Instituto de Biología Molecular y Celular del Cáncer (IBMCC), Universidad de Salamanca-CSIC, Campus Miguel de Unamuno, 37007 Salamanca, Spain; [email protected] 2 Institute of Human Genetics, CNRS, University of Montpellier, 34000 Montpellier, France 3 Department of Biological Hematology, CHU Montpellier, 34295 Montpellier, France 4 Departamento de Microbiología y Genética, Universidad de Salamanca, Campus Miguel de Unamuno, 37007 Salamanca, Spain * Correspondence: [email protected] (S.O.); [email protected] (M.P.S.); Tel.: +34-923-294808 (M.P.S.) Received: 31 January 2020; Accepted: 18 February 2020; Published: 20 February 2020 Abstract: Fidelity in chromosome duplication and segregation is indispensable for maintaining genomic stability and the perpetuation of life. Challenges to genome integrity jeopardize cell survival and are at the root of different types of pathologies, such as cancer. The following three main sources of genomic instability exist: DNA damage, replicative stress, and chromosome segregation defects. In response to these challenges, eukaryotic cells have evolved control mechanisms, also known as checkpoint systems, which sense under-replicated or damaged DNA and activate specialized DNA repair machineries. Cells make use of these checkpoints throughout interphase to shield genome integrity before mitosis. Later on, when the cells enter into mitosis, the spindle assembly checkpoint (SAC) is activated and remains active until the chromosomes are properly attached to the spindle apparatus to ensure an equal segregation among daughter cells. -
S41467-017-00634-0.Pdf
ARTICLE DOI: 10.1038/s41467-017-00634-0 OPEN BRCA2 suppresses replication stress-induced mitotic and G1 abnormalities through homologous recombination Weiran Feng 1,2 & Maria Jasin1,2 Mutations in the tumor suppressor BRCA2 predominantly predispose to breast cancer. Paradoxically, while loss of BRCA2 promotes tumor formation, it also causes cell lethality, although how lethality is triggered is unclear. Here, we generate BRCA2 conditional non- transformed human mammary epithelial cell lines using CRISPR-Cas9. Cells are inviable upon BRCA2 loss, which leads to replication stress associated with under replication, causing mitotic abnormalities, 53BP1 nuclear body formation in the ensuing G1 phase, and G1 arrest. Unexpected from other systems, the role of BRCA2 in homologous recombination, but not in stalled replication fork protection, is primarily associated with supporting human mammary epithelial cell viability, and, moreover, preventing replication stress, a hallmark of pre- cancerous lesions. Thus, we uncover a DNA under replication-53BP1 nuclear body formation- G1 arrest axis as an unanticipated outcome of homologous recombination deficiency, which triggers cell lethality and, we propose, serves as a barrier that must be overcome for tumor formation. 1 Developmental Biology Program, Memorial Sloan Kettering Cancer Center, 1275 York Avenue, New York, NY 10065, USA. 2 Louis V. Gerstner Jr. Graduate School of Biomedical Sciences, Memorial Sloan Kettering Cancer Center, 1275 York Avenue, New York, NY 10065, USA. Correspondence and requests for materials should be addressed to M.J. (email: [email protected]) NATURE COMMUNICATIONS | 8: 525 | DOI: 10.1038/s41467-017-00634-0 | www.nature.com/naturecommunications 1 ARTICLE NATURE COMMUNICATIONS | DOI: 10.1038/s41467-017-00634-0 onoallelic inheritance of a deleterious mutation in Results the BRCA1 or BRCA2 tumor suppressor confers BRCA2 is essential for human mammary MCF10A cell viability. -
Exploiting DNA Replication Stress for Cancer Treatment Tajinder Ubhi1,2 and Grant W
Published OnlineFirst April 9, 2019; DOI: 10.1158/0008-5472.CAN-18-3631 Cancer Review Research Exploiting DNA Replication Stress for Cancer Treatment Tajinder Ubhi1,2 and Grant W. Brown1,2 Abstract Complete and accurate DNA replication is fundamental to associated with such therapies. We discuss how replication cellular proliferation and genome stability. Obstacles that stress modulates the cell-intrinsic innate immune response delay, prevent, or terminate DNA replication cause the phe- and highlight the integration of replication stress with immu- nomena termed DNA replication stress. Cancer cells exhibit notherapies. Together, exploiting replication stress for cancer chronic replication stress due to the loss of proteins that treatment seems to be a promising strategy as it provides a protect or repair stressed replication forks and due to the selective means of eliminating tumors, and with continuous continuous proliferative signaling, providing an exploitable advances in our knowledge of the replication stress response therapeutic vulnerability in tumors. Here, we outline current and lessons learned from current therapies in use, we are and pending therapeutic approaches leveraging tumor-specific moving toward honing the potential of targeting replication replication stress as a target, in addition to the challenges stress in the clinic. Introduction mental. In this review, we provide a summary of the therapies centered on enhancing both endogenous and drug-induced rep- The DNA replication machinery successfully carries out accu- lication stress and discuss the rationales associated with them. We rate genome duplication in the face of numerous obstacles, many also highlight the potential of using replication stress to stimulate of which cause DNA replication stress. -
Exploiting DNA Replication Stress for Cancer Treatment Tajinder Ubhi1,2 and Grant W
Published OnlineFirst April 9, 2019; DOI: 10.1158/0008-5472.CAN-18-3631 Cancer Review Research Exploiting DNA Replication Stress for Cancer Treatment Tajinder Ubhi1,2 and Grant W. Brown1,2 Abstract Complete and accurate DNA replication is fundamental to associated with such therapies. We discuss how replication cellular proliferation and genome stability. Obstacles that stress modulates the cell-intrinsic innate immune response delay, prevent, or terminate DNA replication cause the phe- and highlight the integration of replication stress with immu- nomena termed DNA replication stress. Cancer cells exhibit notherapies. Together, exploiting replication stress for cancer chronic replication stress due to the loss of proteins that treatment seems to be a promising strategy as it provides a protect or repair stressed replication forks and due to the selective means of eliminating tumors, and with continuous continuous proliferative signaling, providing an exploitable advances in our knowledge of the replication stress response therapeutic vulnerability in tumors. Here, we outline current and lessons learned from current therapies in use, we are and pending therapeutic approaches leveraging tumor-specific moving toward honing the potential of targeting replication replication stress as a target, in addition to the challenges stress in the clinic. Introduction mental. In this review, we provide a summary of the therapies centered on enhancing both endogenous and drug-induced rep- The DNA replication machinery successfully carries out accu- lication stress and discuss the rationales associated with them. We rate genome duplication in the face of numerous obstacles, many also highlight the potential of using replication stress to stimulate of which cause DNA replication stress. -
Molecular Regulation of BRD3 in Forward Programming of Megakaryocytes
Molecular regulation of BRD3 in forward programming of Megakaryocytes Maria Isabel Marques Rosa University of Cambridge Newnham College This dissertation is submitted for the degree of Doctor of Philosophy September 2018 Declaration This dissertation is the result of my own work and includes nothing which is the outcome of work done in collaboration except as declared in the Preface and specified in the text. It is not substantially the same as any that I have submitted, or, is being concurrently submitted for a degree or diploma or other qualification at the University of Cambridge or any other University or similar institution except as declared in the Preface and specified in the text. I further state that no substantial part of my dissertation has already been submitted, or, is being concurrently submitted for any such degree, diploma or other qualification at the University of Cambridge or any other University or similar institution except as declared in the Preface and specified in the text. It does not exceed the prescribed word limit for the relevant Degree Committee. Isabel Rosa September 2018 “Quando de lá vimos, é que para lá havíamos de ir” “The moment when one finishes a journey, is when they are truly ready to start.” Ernesto Rosa, my Dad Table of contents Abstract ................................................................................................................................ 1 Introduction ................................................................................................................................ -
Prevention of DNA Replication Stress by CHK1 Leads to Chemoresistance Despite a DNA Repair Defect in Homologous Recombination in Breast Cancer
cells Article Prevention of DNA Replication Stress by CHK1 Leads to Chemoresistance Despite a DNA Repair Defect in Homologous Recombination in Breast Cancer 1, 1, 1 1 Felix Meyer y, Saskia Becker y, Sandra Classen , Ann Christin Parplys , Wael Yassin Mansour 1,2, Britta Riepen 1, Sara Timm 3, Claudia Ruebe 3 , Maria Jasin 4, Harriet Wikman 5 , Cordula Petersen 6, Kai Rothkamm 1 and Kerstin Borgmann 1,* 1 Laboratory of Radiobiology and Experimental Radiooncology, Center of Oncology, University Medical Center Hamburg-Eppendorf, 20246 Hamburg, Germany; [email protected] (F.M.); [email protected] (S.B.); [email protected] (S.C.); [email protected] (A.C.P.); [email protected] (W.Y.M.); [email protected] (B.R.); [email protected] (K.R.) 2 Tumor Biology Department, National Cancer Institute, Cairo University, Cairo 11796, Egypt 3 Department of Radiation Oncology, Saarland University, 66421 Hamburg/Saar, Germany; [email protected] (S.T.); [email protected] (C.R.) 4 Developmental Biology Program, Memorial Sloan Kettering Cancer Center, New York, NY 10065, USA; [email protected] 5 Department of Tumor Biology, University Center Hamburg-Eppendorf, 20246 Hamburg, Germany; [email protected] 6 Department of Radiotherapy and Radiooncology, University Medical Center Hamburg-Eppendorf, 20246 Hamburg, Germany; [email protected] * Correspondence: [email protected]; Tel.: +49-40-74105-3596 These authors contributed equally to the manuscript. y Received: 10 December 2019; Accepted: 14 January 2020; Published: 17 January 2020 Abstract: Chromosomal instability not only has a negative effect on survival in triple-negative breast cancer, but also on the well treatable subgroup of luminal A tumors. -
A Pan-Cancer Transcriptome Analysis Identifies Replication Fork and Innate Immunity Genes As Modifiers of Response to the CHK1 Inhibitor Prexasertib
www.oncotarget.com Oncotarget, 2020, Vol. 11, (No. 3), pp: 216-236 Research Paper A pan-cancer transcriptome analysis identifies replication fork and innate immunity genes as modifiers of response to the CHK1 inhibitor prexasertib Wayne D. Blosser1, Jack A. Dempsey1, Ann M. McNulty1, Xi Rao1, Philip J. Ebert1, Caitlin D. Lowery1, Philip W. Iversen1, Yue Wang Webster1, Gregory P. Donoho1, Xueqian Gong1, Farhana F. Merzoug1, Sean Buchanan1, Karsten Boehnke2, Chunping Yu3, Xin Tian You3, Richard P. Beckmann1, Wenjuan Wu1, Samuel C. McNeely1, Aimee Bence Lin1 and Ricardo Martinez1 1Eli Lilly and Company, Indianapolis, IN, USA 2Eli Lilly and Company, New York, NY, USA 3Eli Lilly and Company, Shanghai, China Correspondence to: Ricardo Martinez, email: [email protected] Keywords: CHK1; prexasertib; replication stress; replication fork; innate immunity Received: September 19, 2019 Accepted: December 02, 2019 Published: January 21, 2020 Copyright: Blosser et al. This is an open-access article distributed under the terms of the Creative Commons Attribution License 3.0 (CC BY 3.0), which permits unrestricted use, distribution, and reproduction in any medium, provided the original author and source are credited. ABSTRACT The combined influence of oncogenic drivers, genomic instability, and/or DNA damage repair deficiencies increases replication stress in cancer. Cells with high replication stress rely on the upregulation of checkpoints like those governed by CHK1 for survival. Previous studies of the CHK1 inhibitor prexasertib demonstrated activity across multiple cancer types. Therefore, we sought to (1) identify markers of prexasertib sensitivity and (2) define the molecular mechanism(s) of intrinsic and acquired resistance using preclinical models representing multiple tumor types. -
CHK1-Targeted Therapy to Deplete DNA Replication-Stressed, P53
Gut Online First, published on April 7, 2017 as 10.1136/gutjnl-2016-312623 GI cancer ORIGINAL ARTICLE CHK1-targeted therapy to deplete DNA replication- Gut: first published as 10.1136/gutjnl-2016-312623 on 7 April 2017. Downloaded from stressed, p53-deficient, hyperdiploid colorectal cancer stem cells Gwenola Manic,1 Michele Signore,2 Antonella Sistigu,3 Giorgio Russo,3,4 Francesca Corradi,1 Silvia Siteni,3,5 Martina Musella,3,6 Sara Vitale,4 Maria Laura De Angelis,2 Matteo Pallocca,7 Carla Azzurra Amoreo,8 Francesca Sperati,9 Simone Di Franco,10 Sabina Barresi,11 Eleonora Policicchio,2,12 Gabriele De Luca,2 Francesca De Nicola,7 Marcella Mottolese,8 Ann Zeuner,2 Maurizio Fanciulli,7 Giorgio Stassi,10 Marcello Maugeri-Saccà,13 Marta Baiocchi,2 Marco Tartaglia,11 Ilio Vitale,1,3 Ruggero De Maria4 ▸ Additional material is ABSTRACT published online only. To view Objective Cancer stem cells (CSCs) are responsible for Significance of this study please visit the journal online (http://dx.doi.org/10.1136/ tumour formation and spreading, and their targeting is gutjnl-2016-312623). required for tumour eradication. There are limited therapeutic options for advanced colorectal cancer What is already known on this subject? (CRC), particularly for tumours carrying RAS-activating ▸ fi Cancer stem cells (CSCs), the subpopulation of For numbered af liations see mutations. The aim of this study was to identify novel end of article. cells driving tumour initiation and spreading, CSC-targeting strategies. are associated with cancer relapse, therapeutic Correspondence to Design To discover potential therapeutics to be resistance and poor patient prognosis thereby Dr. -
ATR-Chk1 Activation Mitigates Replication Stress Caused by Mismatch Repair-Dependent Processing of DNA Damage
ATR-Chk1 activation mitigates replication stress caused by mismatch repair-dependent processing of DNA damage Dipika Guptaa,b, Bo Lina,b,1, Ann Cowanc,d, and Christopher D. Heinena,b,2 aNeag Comprehensive Cancer Center, UConn Health, Farmington, CT 06030-3101; bCenter for Molecular Oncology, UConn Health, Farmington, CT 06030- 3101; cR. D. Berlin Center for Cell Analysis and Modeling, UConn Health, Farmington, CT 06030; and dDepartment of Molecular Biology and Biophysics, UConn Health, Farmington, CT 06030 Edited by Richard D. Kolodner, Ludwig Institute for Cancer Research, La Jolla, CA, and approved December 29, 2017 (received for review December 1, 2017) The mismatch repair pathway (MMR) is essential for removing that the cells continue into a second cell cycle is not clear. Per- DNA polymerase errors, thereby maintaining genomic stability. sistent single-stranded gaps remain after MMR activity that are Loss of MMR function increases mutation frequency and is proposed to become double-strand breaks (DSB) in the next associated with tumorigenesis. However, how MMR is executed S phase, causing the G2 arrest (5). A second model suggests that at active DNA replication forks is unclear. This has important im- the MMR proteins recognize MeG/T and directly recruit proteins plications for understanding how MMR repairs O6-methylguanine/ involved in signaling cell cycle arrest such as Ataxia telangiec- thymidine (MeG/T) mismatches created upon exposure to DNA tasia and Rad3-related (ATR), Ataxia telangiectasia mutated alkylating agents. If MeG/T lesion recognition by MMR initiates (ATM), and the checkpoint kinases Chk1 and Chk2 (3). As the mismatch excision, the reinsertion of a mismatched thymidine dur- MMR proteins respond to MeG/T in the first S phase, it is un- ing resynthesis could initiate futile repair cycles. -
DNA Replication Stress and Chromosomal Instability: Dangerous Liaisons
G C A T T A C G G C A T genes Review DNA Replication Stress and Chromosomal Instability: Dangerous Liaisons Therese Wilhelm 1,2, Maha Said 1 and Valeria Naim 1,* 1 CNRS UMR9019 Genome Integrity and Cancers, Université Paris Saclay, Gustave Roussy, 94805 Villejuif, France; [email protected] (T.W.); [email protected] (M.S.) 2 UMR144 Cell Biology and Cancer, Institut Curie, 75005 Paris, France * Correspondence: [email protected] Received: 11 May 2020; Accepted: 8 June 2020; Published: 10 June 2020 Abstract: Chromosomal instability (CIN) is associated with many human diseases, including neurodevelopmental or neurodegenerative conditions, age-related disorders and cancer, and is a key driver for disease initiation and progression. A major source of structural chromosome instability (s-CIN) leading to structural chromosome aberrations is “replication stress”, a condition in which stalled or slowly progressing replication forks interfere with timely and error-free completion of the S phase. On the other hand, mitotic errors that result in chromosome mis-segregation are the cause of numerical chromosome instability (n-CIN) and aneuploidy. In this review, we will discuss recent evidence showing that these two forms of chromosomal instability can be mechanistically interlinked. We first summarize how replication stress causes structural and numerical CIN, focusing on mechanisms such as mitotic rescue of replication stress (MRRS) and centriole disengagement, which prevent or contribute to specific types of structural chromosome aberrations and segregation errors. We describe the main outcomes of segregation errors and how micronucleation and aneuploidy can be the key stimuli promoting inflammation, senescence, or chromothripsis.