Lower Motor Neuron Circuits and Motor Control 373
Total Page:16
File Type:pdf, Size:1020Kb
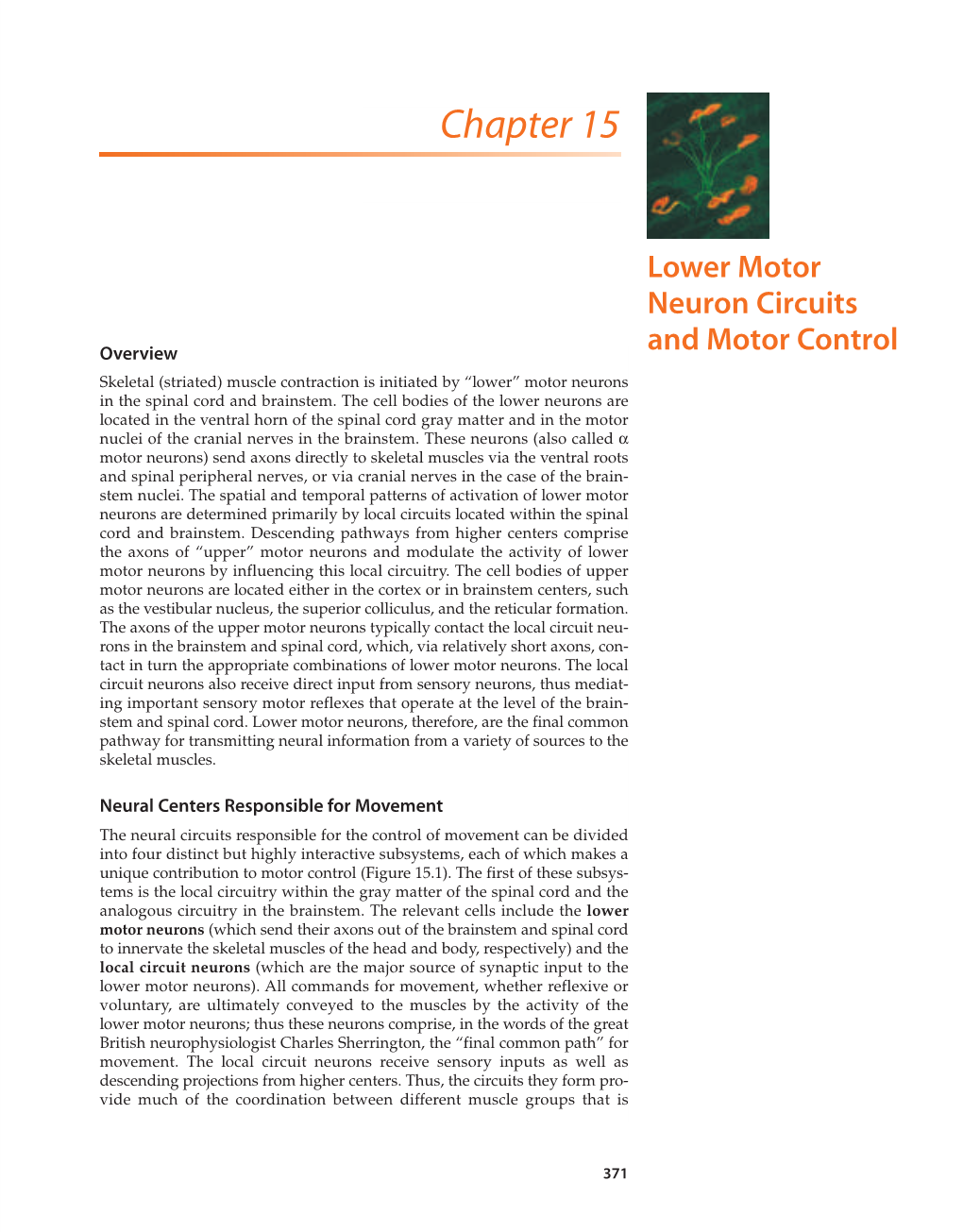
Load more
Recommended publications
-
Of 100 1. the Golgi Tendon Organ Is an Essential Component of Static
1. The Golgi tendon organ is an essential component of static stretching because it A. increases muscle spindle activity in a tight muscle. Rationale A. Golgi tendon organs decrease muscle spindle activity. B. prevents muscles from stretching too far or too fast. Rationale B. Muscle spindles prevent muscles from stretching too far or too fast. C. increases contraction rate in muscle fibers. Rationale C. Holding a stretch creates tension in the muscle, which stimulates the Golgi tendon organ, causes relaxation of an overactive muscle, and allows optimal lengthening of tissue. D. prevents muscle from being placed under excessive tension.(correct) Rationale D. The Golgi tendon organ prevents muscles from being placed under excessive tension (autogenic inhibition). Question Name 1-1 Certification Thinking Skills Foundational Thinking Current Forms 2011 Practice A Primary Reference Chapter 7, Task 1A1 Page 1 of 100 2. Which of the following is the correct force-couple relationship that allows for the upward rotation of the scapula? A. Longus capitus and brachialis Rationale A. The longus capitus concentrically accelerates cervical flexion and lateral flexion, while the brachialis concentrically accelerates elbow flexion. B. Rhomboid minor and anterior scalenes Rationale B. The rhomboid minor concentrically accelerates scapular retraction and downward rotation, while the anterior scalenes concentrically accelerates cervical flexion, rotation, and lateral flexion. C. Sternocleidomastoid and longus coli Rationale C. The sternocleidomastoid concentrically accelerates cervical flexion, rotation, and lateral flexion while the longus coli concentrically accelerate cervical flexion, lateral flexion, and ipsilateral rotation. D. Upper trapezius and lower portions of the serratus anterior (correct) Rationale D. The upper trapezius and the lower portion of the serratus anterior are muscle groups that move together to produce upward rotation of the scapula. -
Primary Lateral Sclerosis, Upper Motor Neuron Dominant Amyotrophic Lateral Sclerosis, and Hereditary Spastic Paraplegia
brain sciences Review Upper Motor Neuron Disorders: Primary Lateral Sclerosis, Upper Motor Neuron Dominant Amyotrophic Lateral Sclerosis, and Hereditary Spastic Paraplegia Timothy Fullam and Jeffrey Statland * Department of Neurology, University of Kansas Medical Center, Kansas, KS 66160, USA; [email protected] * Correspondence: [email protected] Abstract: Following the exclusion of potentially reversible causes, the differential for those patients presenting with a predominant upper motor neuron syndrome includes primary lateral sclerosis (PLS), hereditary spastic paraplegia (HSP), or upper motor neuron dominant ALS (UMNdALS). Differentiation of these disorders in the early phases of disease remains challenging. While no single clinical or diagnostic tests is specific, there are several developing biomarkers and neuroimaging technologies which may help distinguish PLS from HSP and UMNdALS. Recent consensus diagnostic criteria and use of evolving technologies will allow more precise delineation of PLS from other upper motor neuron disorders and aid in the targeting of potentially disease-modifying therapeutics. Keywords: primary lateral sclerosis; amyotrophic lateral sclerosis; hereditary spastic paraplegia Citation: Fullam, T.; Statland, J. Upper Motor Neuron Disorders: Primary Lateral Sclerosis, Upper 1. Introduction Motor Neuron Dominant Jean-Martin Charcot (1825–1893) and Wilhelm Erb (1840–1921) are credited with first Amyotrophic Lateral Sclerosis, and describing a distinct clinical syndrome of upper motor neuron (UMN) tract degeneration in Hereditary Spastic Paraplegia. Brain isolation with symptoms including spasticity, hyperreflexia, and mild weakness [1,2]. Many Sci. 2021, 11, 611. https:// of the earliest described cases included cases of hereditary spastic paraplegia, amyotrophic doi.org/10.3390/brainsci11050611 lateral sclerosis, and underrecognized structural, infectious, or inflammatory etiologies for upper motor neuron dysfunction which have since become routinely diagnosed with the Academic Editors: P. -
Neural Control of Movement: Motor Neuron Subtypes, Proprioception and Recurrent Inhibition
List of Papers This thesis is based on the following papers, which are referred to in the text by their Roman numerals. I Enjin A, Rabe N, Nakanishi ST, Vallstedt A, Gezelius H, Mem- ic F, Lind M, Hjalt T, Tourtellotte WG, Bruder C, Eichele G, Whelan PJ, Kullander K (2010) Identification of novel spinal cholinergic genetic subtypes disclose Chodl and Pitx2 as mark- ers for fast motor neurons and partition cells. J Comp Neurol 518:2284-2304. II Wootz H, Enjin A, Wallen-Mackenzie Å, Lindholm D, Kul- lander K (2010) Reduced VGLUT2 expression increases motor neuron viability in Sod1G93A mice. Neurobiol Dis 37:58-66 III Enjin A, Leao KE, Mikulovic S, Le Merre P, Tourtellotte WG, Kullander K. 5-ht1d marks gamma motor neurons and regulates development of sensorimotor connections Manuscript IV Enjin A, Leao KE, Eriksson A, Larhammar M, Gezelius H, Lamotte d’Incamps B, Nagaraja C, Kullander K. Development of spinal motor circuits in the absence of VIAAT-mediated Renshaw cell signaling Manuscript Reprints were made with permission from the respective publishers. Cover illustration Carousel by Sasha Svensson Contents Introduction.....................................................................................................9 Background...................................................................................................11 Neural control of movement.....................................................................11 The motor neuron.....................................................................................12 Organization -
Interpretation of Sensory Information from Skeletal Muscle Receptors for External Control Milan Djilas
Interpretation of Sensory Information From Skeletal Muscle Receptors For External Control Milan Djilas To cite this version: Milan Djilas. Interpretation of Sensory Information From Skeletal Muscle Receptors For External Control. Automatic. Université Montpellier II - Sciences et Techniques du Languedoc, 2008. English. tel-00333530 HAL Id: tel-00333530 https://tel.archives-ouvertes.fr/tel-00333530 Submitted on 23 Oct 2008 HAL is a multi-disciplinary open access L’archive ouverte pluridisciplinaire HAL, est archive for the deposit and dissemination of sci- destinée au dépôt et à la diffusion de documents entific research documents, whether they are pub- scientifiques de niveau recherche, publiés ou non, lished or not. The documents may come from émanant des établissements d’enseignement et de teaching and research institutions in France or recherche français ou étrangers, des laboratoires abroad, or from public or private research centers. publics ou privés. UNIVERSITE MONTPELLIER II SCIENCES ET TECHNIQUES DU LANGUEDOC T H E S E pour obtenir le grade de DOCTEUR DE L'UNIVERSITE MONTPELLIER II Formation doctorale: SYSTEMES AUTOMATIQUES ET MICROELECTRONIQUES Ecole Doctorale: INFORMATION, STRUCTURES ET SYSTEMES présentée et soutenue publiquement par Milan DJILAS le 13 octobre 2008 Titre: INTERPRETATION DES INFORMATIONS SENSORIELLES DES RECEPTEURS DU MUSCLE SQUELETTIQUE POUR LE CONTROLE EXTERNE INTERPRETATION OF SENSORY INFORMATION FROM SKELETAL MUSCLE RECEPTORS FOR EXTERNAL CONTROL JURY Jacques LEVY VEHEL Directeur de Recherches, INRIA Rapporteur -
Inside Your Brain You and Your Brain
Inside your brain You and your brain Many simple and complex psychological functions are mediated by multiple brain regions and, at the same time, a single brain area may control many psychological functions. CC BY Illustration by Bret Syfert 1. Cortex: The thin, folded structure on the outside surface of the brain. 2. Cerebral hemispheres: The two halves of the brain, each of which controls and receives information from the opposite side of the body. 3. Pituitary gland: The ‘master gland’ of the body, which releases hormones that control growth, blood pressure, the stress response and the function of the sex organs. 4. Substantia nigra: The ‘black substance’ contains cells that produce the neurotransmitter dopamine and the pigment melatonin, giving it a black appearance. 5. Hypothalamus: The interface between the brain and pituitary gland. It controls the production and release of hormones. 6. Spinal cord: A large bundle of millions of nerve fibres and neuronal cells, which carries information back and forth between the brain and the body. 7. Medulla oblongata: Controls vital involuntary functions such as breathing and heart rate. 8. Cerebellum: The ‘little brain’ that controls balance and coordinates movements. It’s normally required for learning motor skills, such as riding a bike, and is involved in thought processes. 9. Cranial nerve nuclei: Clusters of neurons in the brain stem. Their axons form the cranial nerves. Your brain underpins who you are. It stores your knowledge and memories, gives you the capacity for thought and emotion, and enables you to control your body. The brain is just one part of the nervous system. -
Chapter 8 Nervous System
Chapter 8 Nervous System I. Functions A. Sensory Input – stimuli interpreted as touch, taste, temperature, smell, sound, blood pressure, and body position. B. Integration – CNS processes sensory input and initiates responses categorizing into immediate response, memory, or ignore C. Homeostasis – maintains through sensory input and integration by stimulating or inhibiting other systems D. Mental Activity – consciousness, memory, thinking E. Control of Muscles & Glands – controls skeletal muscle and helps control/regulate smooth muscle, cardiac muscle, and glands II. Divisions of the Nervous system – 2 anatomical/main divisions A. CNS (Central Nervous System) – consists of the brain and spinal cord B. PNS (Peripheral Nervous System) – consists of ganglia and nerves outside the brain and spinal cord – has 2 subdivisions 1. Sensory Division (Afferent) – conducts action potentials from PNS toward the CNS (by way of the sensory neurons) for evaluation 2. Motor Division (Efferent) – conducts action potentials from CNS toward the PNS (by way of the motor neurons) creating a response from an effector organ – has 2 subdivisions a. Somatic Motor System – controls skeletal muscle only b. Autonomic System – controls/effects smooth muscle, cardiac muscle, and glands – 2 branches • Sympathetic – accelerator “fight or flight” • Parasympathetic – brake “resting and digesting” * 4 Types of Effector Organs: skeletal muscle, smooth muscle, cardiac muscle, and glands. III. Cells of the Nervous System A. Neurons – receive stimuli and transmit action potentials -
ALS and Other Motor Neuron Diseases Can Represent Diagnostic Challenges
Review Article Address correspondence to Dr Ezgi Tiryaki, Hennepin ALS and Other Motor County Medical Center, Department of Neurology, 701 Park Avenue P5-200, Neuron Diseases Minneapolis, MN 55415, [email protected]. Ezgi Tiryaki, MD; Holli A. Horak, MD, FAAN Relationship Disclosure: Dr Tiryaki’s institution receives support from The ALS Association. Dr Horak’s ABSTRACT institution receives a grant from the Centers for Disease Purpose of Review: This review describes the most common motor neuron disease, Control and Prevention. ALS. It discusses the diagnosis and evaluation of ALS and the current understanding of its Unlabeled Use of pathophysiology, including new genetic underpinnings of the disease. This article also Products/Investigational covers other motor neuron diseases, reviews how to distinguish them from ALS, and Use Disclosure: Drs Tiryaki and Horak discuss discusses their pathophysiology. the unlabeled use of various Recent Findings: In this article, the spectrum of cognitive involvement in ALS, new concepts drugs for the symptomatic about protein synthesis pathology in the etiology of ALS, and new genetic associations will be management of ALS. * 2014, American Academy covered. This concept has changed over the past 3 to 4 years with the discovery of new of Neurology. genes and genetic processes that may trigger the disease. As of 2014, two-thirds of familial ALS and 10% of sporadic ALS can be explained by genetics. TAR DNA binding protein 43 kDa (TDP-43), for instance, has been shown to cause frontotemporal dementia as well as some cases of familial ALS, and is associated with frontotemporal dysfunction in ALS. Summary: The anterior horn cells control all voluntary movement: motor activity, res- piratory, speech, and swallowing functions are dependent upon signals from the anterior horn cells. -
Innovations Present in the Primate Interneuron Repertoire
Article Innovations present in the primate interneuron repertoire https://doi.org/10.1038/s41586-020-2781-z Fenna M. Krienen1,2 ✉, Melissa Goldman1,2, Qiangge Zhang2,3, Ricardo C. H. del Rosario2, Marta Florio1,2, Robert Machold4, Arpiar Saunders1,2, Kirsten Levandowski2,3, Heather Zaniewski2,3, Received: 19 July 2019 Benjamin Schuman4, Carolyn Wu3, Alyssa Lutservitz1,2, Christopher D. Mullally1,2, Nora Reed1,2, Accepted: 1 July 2020 Elizabeth Bien1,2, Laura Bortolin1,2, Marian Fernandez-Otero2,5, Jessica D. Lin2, Alec Wysoker2, James Nemesh2, David Kulp2, Monika Burns5, Victor Tkachev6,7,8, Richard Smith9,10, Published online: xx xx xxxx Christopher A. Walsh9,10, Jordane Dimidschstein2, Bernardo Rudy4,11, Leslie S. Kean6,7,8, Check for updates Sabina Berretta5,12,13, Gord Fishell2,14, Guoping Feng2,3 & Steven A. McCarroll1,2 ✉ Primates and rodents, which descended from a common ancestor around 90 million years ago1, exhibit profound diferences in behaviour and cognitive capacity; the cellular basis for these diferences is unknown. Here we use single-nucleus RNA sequencing to profle RNA expression in 188,776 individual interneurons across homologous brain regions from three primates (human, macaque and marmoset), a rodent (mouse) and a weasel (ferret). Homologous interneuron types—which were readily identifed by their RNA-expression patterns—varied in abundance and RNA expression among ferrets, mice and primates, but varied less among primates. Only a modest fraction of the genes identifed as ‘markers’ of specifc interneuron subtypes in any one species had this property in another species. In the primate neocortex, dozens of genes showed spatial expression gradients among interneurons of the same type, which suggests that regional variation in cortical contexts shapes the RNA expression patterns of adult neocortical interneurons. -
Fine Structure of the Receptors at the Myotendinous Junction of Human Extraocular Muscles
Histol Histopath (1 988) 3: 103-113 Histology and Fine structure of the receptors at the myotendinous junction of human extraocular muscles A. Sodii, M. Corsii, M.S. Faussone Pellegrini2and G. Salvii 'Eye Clinic, Chair of Physiopathological Optics and Departrnent of Hurnan Anatorny and Histology, Section of Histology, University of Florence, ltaly Summary. The myotendinous junction of the human lntroduction extraocular muscles was studied by electron microscopy. Some peculiar receptorial structures have been found in The proprioceptors known as tendon organs were first the majority of the samples examined. These structures identified by Golgi in 1880 in skeletal muscles. They were are very small and consist of 1) the terminal portion of first described at electron microscope level by Merrillees one muscle fibre, 2) the tendon into which it inserts and in 1962 and later by other authors (Schoultz and Swett, y), within the tendon, a rich nerve arborization, whose 1972, 1974; Barker, 1974: Zelena and Soukup, 1977; branches are always very close to the rnuscle component. Soukup and Zelena, 1985; Ovalle and Dow, 1983). In the Only one discontinuous layer, made up of tlat cells. extraocular muscles (EOM) the presence of tendon which lack a basa1 lamina and often show pinocytotic organs was first excluded by Golgi himself, but further vesicles, encapsules every musculo-tendinous complex. investigations (Dogiel. 1906: Loffredo-Sampaolo, 1952; The tendinous component consists of amorphous ground Bonavolonta, 1956, 1958) led to their identification and substance of different electron density. of collagen and description at light microscopy level in several animal elastic fibres and is divided in compartments by ramified species. -
Skeletal Muscle Physiology
This document was created by Alex Yartsev ([email protected]); if I have used your data or images and forgot to reference you, please email me. Skeletal Muscle Physiology First of all, which muscle is which - Skeletal muscle: o Well-developed cross-striations o Does not contract in absence of a nerve stimulus o The individual muscle fibers DO NOT connect functionally or anatomically (i.e. they don’t form a single sheet of cells, and one fiber’s action potential wont get transmitted to the next) o Generally, skeletal muscle is under voluntary control - Cardiac muscle: o Also has cross-striations o Is functionally syncytial: cells are connected well enough to conduct action potentials to one another o Can contract on its own, without stimulus (but this is under some control via the autonomic nervous system, which modulates its activity) - Smooth muscle: o Has no cross-striations o Two broad types: . VISCERAL or “unitary” smooth muscle: Functionally syncytial, action potentials propagate from cell to cell Contains pacemakers which discharge irregularly, but remains under control of the autonomic nervous system Found in most hollow viscera . MULTI-UNIT SMOOTH MUSCLE Found in the eye and some other locations Does NOT activate spontaneously SKELETAL MUSCLE ORGANIZATION - Each muscle is a bundle of fibers - Each fiber is a long, multinucleated single cell - Each fiber is surrounded by a SARCOLEMMA- the cell membrane - There are NO SYNCYTIAL BRIDGES between the cells. When one cell goes off, the others don’t follow. TRANSVERSE TUBULES: T-tubules, invaginations of SARCOLEMMA: the muscle cell membrane the sarcolemma, they form part of the T-system; the space inside is an extension of the extracellular space. -
Back-To-Basics: the Intricacies of Muscle Contraction
Back-to- MIOTA Basics: The CONFERENCE OCTOBER 11, Intricacies 2019 CHERI RAMIREZ, MS, of Muscle OTRL Contraction OBJECTIVES: 1.Review the anatomical structure of a skeletal muscle. 2.Review and understand the process and relationship between skeletal muscle contraction with the vital components of the nervous system, endocrine system, and skeletal system. 3.Review the basic similarities and differences between skeletal muscle tissue, smooth muscle tissue, and cardiac muscle tissue. 4.Review the names, locations, origins, and insertions of the skeletal muscles found in the human body. 5.Apply the information learned to enhance clinical practice and understanding of the intricacies and complexity of the skeletal muscle system. 6.Apply the information learned to further educate clients on the importance of skeletal muscle movement, posture, and coordination in the process of rehabilitation, healing, and functional return. 1. Epithelial Four Basic Tissue Categories 2. Muscle 3. Nervous 4. Connective A. Loose Connective B. Bone C. Cartilage D. Blood Introduction There are 3 types of muscle tissue in the muscular system: . Skeletal muscle: Attached to bones of skeleton. Voluntary. Striated. Tubular shape. Cardiac muscle: Makes up most of the wall of the heart. Involuntary. Striated with intercalated discs. Branched shape. Smooth muscle: Found in walls of internal organs and walls of vascular system. Involuntary. Non-striated. Spindle shape. 4 Structure of a Skeletal Muscle Skeletal Muscles: Skeletal muscles are composed of: • Skeletal muscle tissue • Nervous tissue • Blood • Connective tissues 5 Connective Tissue Coverings Connective tissue coverings over skeletal muscles: .Fascia .Tendons .Aponeuroses 6 Fascia: Definition: Layers of dense connective tissue that separates muscle from adjacent muscles, by surrounding each muscle belly. -
A System for Studying Mechanisms of Neuromuscular Junction Development and Maintenance Valérie Vilmont1,‡, Bruno Cadot1, Gilles Ouanounou2 and Edgar R
© 2016. Published by The Company of Biologists Ltd | Development (2016) 143, 2464-2477 doi:10.1242/dev.130278 TECHNIQUES AND RESOURCES RESEARCH ARTICLE A system for studying mechanisms of neuromuscular junction development and maintenance Valérie Vilmont1,‡, Bruno Cadot1, Gilles Ouanounou2 and Edgar R. Gomes1,3,*,‡ ABSTRACT different animal models and cell lines (Chen et al., 2014; Corti et al., The neuromuscular junction (NMJ), a cellular synapse between a 2012; Lenzi et al., 2015) with the hope of recapitulating some motor neuron and a skeletal muscle fiber, enables the translation of features of neuromuscular diseases and understanding the triggers chemical cues into physical activity. The development of this special of one of their common hallmarks: the disruption of the structure has been subject to numerous investigations, but its neuromuscular junction (NMJ). The NMJ is one of the most complexity renders in vivo studies particularly difficult to perform. studied synapses. It is formed of three key elements: the lower motor In vitro modeling of the neuromuscular junction represents a powerful neuron (the pre-synaptic compartment), the skeletal muscle (the tool to delineate fully the fine tuning of events that lead to subcellular post-synaptic compartment) and the Schwann cell (Sanes and specialization at the pre-synaptic and post-synaptic sites. Here, we Lichtman, 1999). The NMJ is formed in a step-wise manner describe a novel heterologous co-culture in vitro method using rat following a series of cues involving these three cellular components spinal cord explants with dorsal root ganglia and murine primary and its role is basically to ensure the skeletal muscle functionality.