(Clostridium Welchii-Type) on Neuromuscular Transmission in the Mouse
Total Page:16
File Type:pdf, Size:1020Kb
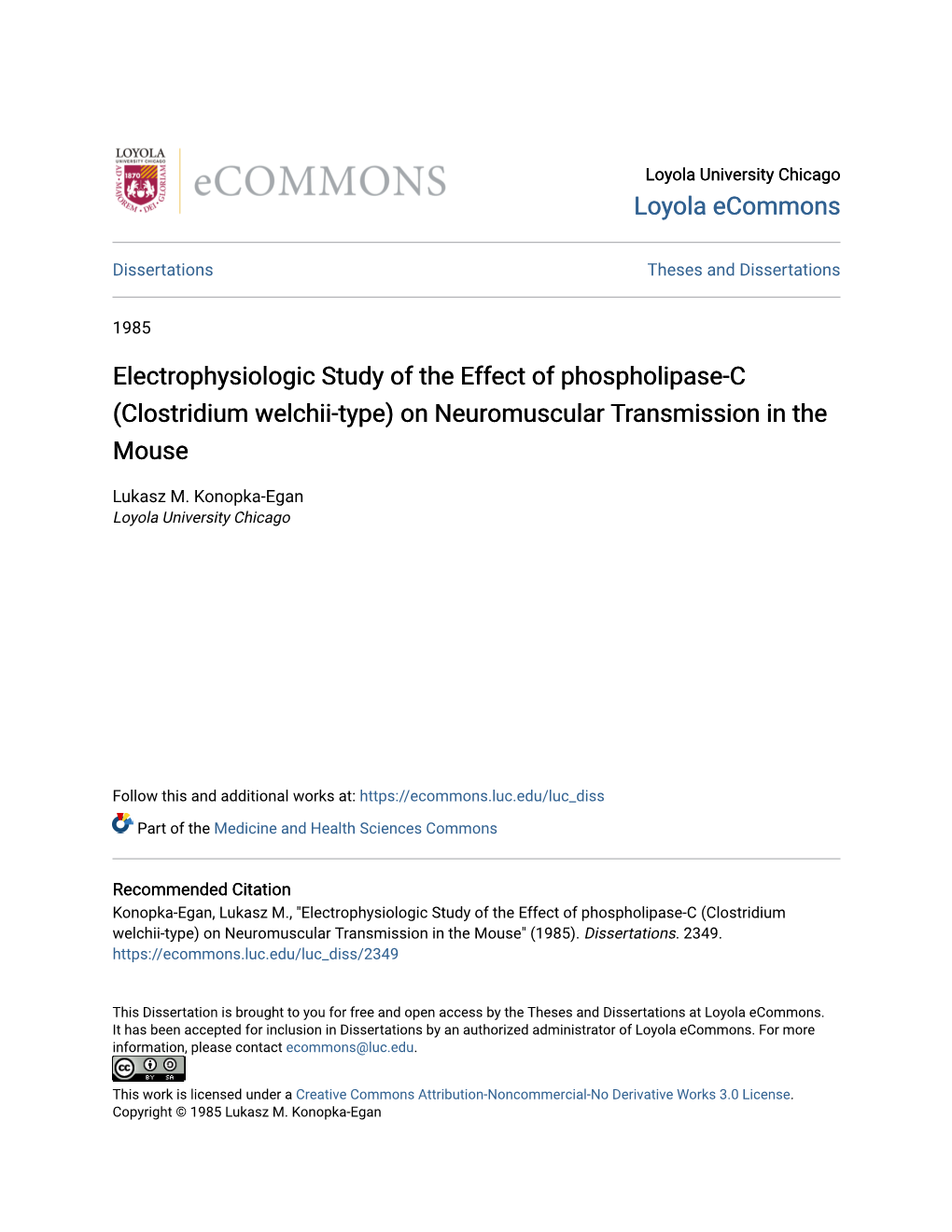
Load more
Recommended publications
-
Medical Bacteriology
LECTURE NOTES Degree and Diploma Programs For Environmental Health Students Medical Bacteriology Abilo Tadesse, Meseret Alem University of Gondar In collaboration with the Ethiopia Public Health Training Initiative, The Carter Center, the Ethiopia Ministry of Health, and the Ethiopia Ministry of Education September 2006 Funded under USAID Cooperative Agreement No. 663-A-00-00-0358-00. Produced in collaboration with the Ethiopia Public Health Training Initiative, The Carter Center, the Ethiopia Ministry of Health, and the Ethiopia Ministry of Education. Important Guidelines for Printing and Photocopying Limited permission is granted free of charge to print or photocopy all pages of this publication for educational, not-for-profit use by health care workers, students or faculty. All copies must retain all author credits and copyright notices included in the original document. Under no circumstances is it permissible to sell or distribute on a commercial basis, or to claim authorship of, copies of material reproduced from this publication. ©2006 by Abilo Tadesse, Meseret Alem All rights reserved. Except as expressly provided above, no part of this publication may be reproduced or transmitted in any form or by any means, electronic or mechanical, including photocopying, recording, or by any information storage and retrieval system, without written permission of the author or authors. This material is intended for educational use only by practicing health care workers or students and faculty in a health care field. PREFACE Text book on Medical Bacteriology for Medical Laboratory Technology students are not available as need, so this lecture note will alleviate the acute shortage of text books and reference materials on medical bacteriology. -
Unit 5 General Techniques of Detection and Enumeration
General Techniques of UNIT 5 GENERAL TECHNIQUES OF Detection and Enumeration DETECTION AND ENUMERATION of Micro-Organisms in Food OF MICRO-ORGANISMS IN FOOD Structure 5.0 Objectives 5.1 Introduction 5.2 Microbiological Media 5.2.1 Types of Media 5.2.2 Preparation of Media 5.3 Enumeration Procedures 5.3.1 Direct Microscopic Count (DMC) 5.3.2 Standard Plate Count (SPC) 5.3.3 Spiral Plate Count 5.3.4 Dye Reduction Tests 5.3.5 Most Probable Number (MPN) Method 5.4 Pure Culture Method 5.5 Microscopic Examination of the Bacterial Culture 5.5.1 Simple Staining 5.5.2 Negative Staining 5.5.3 Gram Staining 5.5.4 Endospore Staining 5.6 Let Us Sum Up 5.7 Keywords 5.8 Suggested Further Reading 5.9 Terminal Questions 5.10 Answers To Check Your Progress Exercises 5.0 OBJECTIVES After reading this Unit, you will be able to: • identify various types of microbiological media and their method of preparation; • describe different enumeration techniques of micro-organisms; • prepare pure culture of micro-organisms from mixed population; and • identify micro-organisms on the basis of microscopic observation. 5.1 INRODUCTION Food microbiology is the branch of microbiology that deals with various types of micro-organisms occurring in the food products. These range from the beneficial micro-organisms to the harmful ones that contaminate foods and lead to its spoilage or disease out breaks by utilizing food as a vector for their spread causing food borne illness. Micro organisms may be added deliberately in the preparation of food products and in this case they are called the starter cultures that play an important role in determining the taste, texture and other properties of a food product. -
1Thisinvestigationwassupported
[CANCER RESEARCH 33, 1889-1897, August 1973] Distribution and Regulation of Deoxythymidine Kinase Activity in Differentiating Cells of Mammalian Intestines' Josephine S. Salser and M. Earl Balis Division of Cell Metabolism, Sloan-Kettering Institute for Cancer Research, New York, New York 10021 SUMMARY TdR kinase (ATP-thymidine 5'-phosphotransferase, EC 2.71.21) activity, which decreases markedly when epithelial The distribution of deoxythymidine kinase activity has cells leave the crypts of rat jejunum on the lower one-third of been reexamined in rat and human intestinal mucosa human colonic mucosa, is generally associated with rapid separated by a planing technique into cells in different growth and increased DNA synthesis ( I2, 32). The pen stages of differentiation. When epithelial cells leave the odicity of this enzyme during the mitotic cycle is well crypts, a decrease in kinase activity was observed in both established but the specific mechanisms that tie the levels of resuspended pellet and supernatant fractions. Commercial kinase activity to the rates of DNA synthesis and cell preparations of phospholipase C added to the assay system division are far from well understood. Synthesis of new resulted in remarkable stimulation of kinase activity, an enzyme rather than change in turnover rate of enzyme effect that was more pronounced in extracts from rat tissues protein could explain the sharp increases in activity ob and in pellet fractions of all the tissues studied. Although served in regenerating liver (2, 9, 28), the S phase of pellet activity in preparations from human mucosa was synchronized cultures of mammalian cells ( 1, 26, 29, 39), primarily associated with the nuclear fraction, significant and virus-infected tissue culture cells (24, 25, 33). -
(12) Patent Application Publication (10) Pub. No.: US 2016/0244489 A1 MASIGNAN Et Al
US 20160244489A1 (19) United States (12) Patent Application Publication (10) Pub. No.: US 2016/0244489 A1 MASIGNAN et al. (43) Pub. Date: Aug. 25, 2016 (54) PROTEINS AND NUCLEICACIDS FROM application No. 1 1/884,812, filed on Mar. 26, 2008, MENINGITIS/SEPSIS-ASSOCATED now Pat. No. 8,758,764, filed as application No. PCT/ ESCHERICHA COL US2006/005913 on Feb. 17, 2006. (71) Applicants: GlaxoSmithKline Biologicals SA, (60) Provisional application No. 60/712,720, filed on Aug. Rixensart (BE); J. Craig Venter 29, 2005, provisional application No. 60/654,632, Institute, Inc., Rockville, MD (US) filed on Feb. 18, 2005. (72) Inventors: Vega MASIGNANI, Siena (IT): Danilo Gomes MORIEL, Monteriggioni (IT): Publication Classification Francesco BERLANDA SCORZA, Trento (IT): Nathalie NORAIS, Siena (51) Int. Cl. (IT): Maria Rita FONTANA, Siena C07K I4/245 (2006.01) (IT); Mariagrazia PIZZA, Siena (IT): A6139/08 (2006.01) Laura SERINO, Monticiano (IT): C07K 6/2 (2006.01) Herve TETTELIN, Gaithersburg, MD (52) U.S. Cl. (US) CPC ........... C07K 14/245 (2013.01); C07K 16/1232 (73) Assignees: GlaxoSmithKline Biologicals SA, (2013.01); A61 K39/0258 (2013.01); A61 K Rixensart (BE); J. Craig Venter 2039/523 (2013.01) Institute, Inc., Rockville, MD (US) (21) Appl. No.: 15/150,204 (57) ABSTRACT (22) Filed: May 9, 2016 Disclosed herein are various open reading frames from a Related U.S. Application Data strain of E. coli responsible for neonatal meningitis (MNEC), (62) Division of application No. 14/293,967, filed on Jun. 2, and a Subset of these that is of particular interest for preparing 2014, now Pat. -
Phospholipase C Expressed in Pichia Pastoris
PHOSPHOLIPASE C EXPRESSED IN PICHIA PASTORIS New specifications prepared at the 69th JECFA (2008), published in FAO JECFA Monographs 5 (2008). An ADI "not specified" was established at the 69th JECFA (2008). SYNONYMS Phospholipase C; lecithinase C; lipophosphodiesterase C; phosphatidase C SOURCES Phospholipase C is produced by submerged fed-batch fermentation of a genetically modified strain of Pichia pastoris which contains the phospholipase C gene derived from a soil sample. The enzyme is recovered from the fermentation broth. The recovery process includes the separation of cellular biomass, clarification, ultrafiltration, diafiltration, and polish filtration. The final product is formulated using food-grade stabilizing and preserving agents and is standardized to the desired activity. Active principles Phospholipase C Systematic names and Phosphatidylcholine cholinephosphohydrolase; EC 3.1.4.3; CAS No. numbers 9001-86-9 Reactions catalysed Hydrolysis of phosphodiester bonds at the sn-3 position in glycerophospholipids including phosphatidylcholine, phosphatidylethanolamine, and phosphatidylserine to yield 1,2- diacylglycerol and the corresponding phosphate esters Secondary enzyme No significant levels of secondary enzyme activities. activities DESCRIPTION Yellow to brown liquid FUNCTIONAL USES Enzyme preparation. Used in refining vegetable oils intended for human consumption. GENERAL Must conform to the latest edition of the JECFA General SPECIFICATIONS Specifications and Considerations for Enzyme Preparations Used in Food Processing. CHARACTERISTICS IDENTIFICATION Phospholipase C activity The sample shows phospholipase C activity. See description under TESTS. TESTS Enzyme activity Principle Phospholipase C catalyses the hydrolysis of phosphatidylcholine to 1,2-diacylglycerol and phosphorylcholine. Phosphorylcholine is subsequently titrated with potassium hydroxide. The activity of phospholipase C is determined by measuring the rate of consumption of potassium hydroxide required to maintain pH 7.3 at 37o. -
(12) United States Patent (10) Patent No.: US 8,758,764 B2 Masignani Et Al
US008758764B2 (12) United States Patent (10) Patent No.: US 8,758,764 B2 Masignani et al. (45) Date of Patent: Jun. 24, 2014 (54) PROTEINS AND NUCLEICACIDS FROM WO WO-O2/O771.83 10, 2002 MENINGITIS/SEPSIS-ASSOCATED WO WO-2004/005535 A2 1/2004 ESCHERICHLA COLI WO WO-2005, O76O10 8, 2005 (75) Inventors: Vega Masignani, Siena (IT); Danilo OTHER PUBLICATIONS Gomes Moriel, Monteriggioni (IT): Houghten et al. (New Approaches to Immunization, Vaccines36, Francesco Berlanda Scorza, Trento Cold Spring Harbor Laboratory, p. 21-25, 1986.* (IT): Nathalie Norais, Siena (IT): Maria McGuinness et al. (Lancet 337: 514-517, Mar. 1991.* Rita Fontana, Siena (IT); Mariagrazia McGuinness et al. (Mol. Microbiol. 7:505-514, Feb. 1993).* Pizza, Siena (IT): Laura Serino, Accession No. Q46837 2002 (or Q2M9M2 or Q46838 or Q6BF5) or Monticiano (IT): Herve Tettelin, Science 277:1453-1474(1997).* Gaithersburg, MD (US) EMBL accession No. AJ617685 (Created on May 11, 2004), "Escherichia coli pathogenicity island V. strain 536.” (73) Assignees: Novartis Vaccines and Diagnostics Srl, UniProt accession No. Q46837 (Integrated on Apr. 16, 2002). “Puta Siena (IT); J. Craig Venter Institute, tive lipprotein acflD homolog.” Rockville, MD (US) Hughes et al. (Aug. 19, 1994). “Sequence analysis of the Vibrio cholerae acfD gene reveals the presence of an overlapping reading (*) Notice: Subject to any disclaimer, the term of this frame, orf7, which encodes a protein that shares sequence similarity patent is extended or adjusted under 35 to the FIiA and FIiC products of Salmonella,", Gene 146(1): 79-82. European Search Report mailed Dec. 23, 2008, for EP patent appli U.S.C. -
Bacillus Cereus
JOURNAL OF ßACTERIOLOGY, Feb. 1989, p. 744-753 Vol. 171, No. 2 0021-9193/89/020744-10$02.00/0 Copyright © 1989, American Society for Microbiology A Bacillus cereus Cytolytic Determinant, Cereolysin AB, Which Comprises the Phospholipase C and Sphingomyelinase Genes: Nucleotide Sequence and Genetic Linkage MICHAEL S. GILMORE,h ARMANDO L. CRUZ-RODZ,1 MICHAELA LEIMEISTER-WÄCHTER,2 2 JÜRGEN KREFT,2 AND WERNER GOEBEL Department of Microbiology and lmmunology, University of Oklahoma Hea/th Seiences Center, P.O. Box 26901, Oklahoma City, Oklahoma 73190, 1 and Institut für Genetik und Mikrobiologie der Universität Würzburg, D-8700 Würzburg, I I Röntgenring, Federal Republic ofGermany2 Received 8 July 1988/Accepted 1 November 1988 A cloned cytolytic determinant from the genome of Bacillus cereus GP-4 has been characterized at the molecular Ievel. Nucleotide sequence determination revealed the presence of two open reading frames. 8oth open reading frames were found by deletion and complementation analysis to be necessary for expression of the hemolytic phenotype by Bacillus subtilis and Escherichia coli hosts. The 5' open reading frame was found to be nearly identical to a recently reported phospholipase C gene derived from a mutant B. cereus strain which overexpresses the respective protein, and it conferred a lecithinase-positive phenotype to the B. subtilis host. The 3' open reading frame encoded a sphingomyelinase. The two tandemly encoded activities, phospholipase C and sphingomyelinase, constitute a biologically functional cytolytic determinant of B. cereus termed cereolysin AB. Bacillus cereus, a common soil saprophyte, has been phospholipase C and sphingomyelinase activity, respec recognized as an opportunistic pathogen of increasing im tively. -
Isolation, Characterization of Phytase Producing Bacillus Sps Nbtrs6 from the Rhizospere Soil of Nbt Cotton Field
Int.J.Curr.Microbiol.App.Sci (2013) 2(10): 142-149 ISSN: 2319-7706 Volume 2 Number 10 (2013) pp. 142-149 http://www.ijcmas.com Original Research Article Isolation, characterization of phytase producing Bacillus sps NBtRS6 from the rhizospere soil of NBt cotton field K.Ushasri*, P.Sivaragini and .K.Vijayalakshmi Department of Applied Microbiology, S.P.M.V.V. Tirupati - 517 502 A. P. India *Corresponding author A B S T R A C T K e y w o r d s Soil samples of Bt Rhizosphere were collected from NBt cotton growing area of Andhra Pradesh, India and was used as a source material for isolation and Immobilized screening of phytase producing bacteria. 21 Bacteria were isolated from NBt P; Rhizosphere. Phytase enzyme activity of the cultures was screened on Modified Rhizosphere; phytase solubulizing medium (MPSM). The result inferred that six isolates Phosphatases; NBtRS1 to NBtRS6 were strongly positive in enzyme activity than six of other Phospho- microorganisms while nine isolates were found negative and among all ,Isolate rylation; NBtRS6 produced significantly higher phytase yield than other isolates and was chosen for species identification. Preliminary identification by microscopic and Tropical phosphates Soils. biochemical tests identified the isolate NBtRS6 as Bacillus sp and designated as Bacillus sp NBtRS6. Introduction In nature, phosphorus cycle plays an (both organic and inorganic) to a form important role in the survival of living accessible to the plants, like organisms (Stewart and Mckercher, 1982). orthophosphate, is an important trait for a There are two components of P in soil, PGPB for increasing plant yields. -
ID 8 | Issue No: 4.1 | Issue Date: 01.03.16 | Page: 1 of 27 © Crown Copyright 2016 Identification of Clostridium Species
UK Standards for Microbiology Investigations Identification of Clostridium species Issued by the Standards Unit, Microbiology Services, PHE Bacteriology – Identification | ID 8 | Issue no: 4.1 | Issue date: 01.03.16 | Page: 1 of 27 © Crown copyright 2016 Identification of Clostridium species Acknowledgments UK Standards for Microbiology Investigations (SMIs) are developed under the auspices of Public Health England (PHE) working in partnership with the National Health Service (NHS), Public Health Wales and with the professional organisations whose logos are displayed below and listed on the website https://www.gov.uk/uk- standards-for-microbiology-investigations-smi-quality-and-consistency-in-clinical- laboratories. SMIs are developed, reviewed and revised by various working groups which are overseen by a steering committee (see https://www.gov.uk/government/groups/standards-for-microbiology-investigations- steering-committee). The contributions of many individuals in clinical, specialist and reference laboratories who have provided information and comments during the development of this document are acknowledged. We are grateful to the Medical Editors for editing the medical content. For further information please contact us at: Standards Unit Microbiology Services Public Health England 61 Colindale Avenue London NW9 5EQ E-mail: [email protected] Website: https://www.gov.uk/uk-standards-for-microbiology-investigations-smi-quality- and-consistency-in-clinical-laboratories UK Standards for Microbiology Investigations are produced in association with: Logos correct at time of publishing. Bacteriology – Identification | ID 8 | Issue no: 4.1 | Issue date: 01.03.16 | Page: 2 of 27 UK Standards for Microbiology Investigations | Issued by the Standards Unit, Public Health England Identification of Clostridium species Contents ACKNOWLEDGMENTS ......................................................................................................... -
By 4 4.E. V. 4-1-1
Jan. , 1974 R. H. MOYER ET Al 3,783, 105 APPARATUS FOR ASSAY ING ENZYME ACTIWITY Filed Jan. 27, 197l NWERTORS RUDOLPH. H. MOYER DONALD J S BBETT by 4 4.e. v. 4-1-1- AT TORNEY United States Patent Office Patented Jan.3,783,105 1, 1974 2 3,783,105 Hepatobiliary diseases APPARATUS FOR ASSAYNG ENZYME ACTIVETY Rudolph H. Moyer, West Covina, and Donald J. Sibbett, In application of blood or serum enzyme activity tests Cucamonga, Calif., assignor to Geomet, Incorporated, to the diagnosis of disorders of the liver and its duct sys Rockville, Md. tems, it is possible only in relatively few cases to dis Filed Jan 27, 1971, ser, No. 110,185 criminate between the possible sources of the enzymatic The portion of the term of the patent subsequent to inbalance indicative of specific pathological conditions. May 1. is islaimed However, the following enzymes are considered particu U.S. C. 195-127 VW 11 Claims larly useful in support of diagnosis of such diseases: alka line phosphatase, lactic dehydrogenase, glutamic oxalacetic 10 transaminase, isocitric dehydrogenase, sorbitol dehydro ABSTRACT OF THE DISCLOSURE genase, leucine aminopetidase, aldolase, and 5'-nucleo Improved method and apparatus for quantitating the tidase among others. activities of a wide selection of enzymes present in bio- The following table shows some of the diseases which logical fluids, using a spot test technique. The test ultilizes specifically give rise to considerably increased levels of enzyme standards freeze-dryed on transparent membranes 15 these enzymes and the disorders indicated. assembled together with but separated from reagents, including substrates, dyes, and cofactors, which are freeze- E. -
Download (PDF)
Supplemental Table S1. Comparison of the annotation results for ortholog clusters T T T T Supplemental Table S1. Comparison of the annotation results derived for ortholog clusters from derived from the the B1-EBB1 -andEB HKI454 andgenomes. HKI454 genomes. -
The Toxigenic and Hemolytic Activity of Clostridium Perfringens Isolated from Foods Feces and Soil
University of Montana ScholarWorks at University of Montana Graduate Student Theses, Dissertations, & Professional Papers Graduate School 1968 The toxigenic and hemolytic activity of Clostridium perfringens isolated from foods feces and soil Judith Ann Schulze The University of Montana Follow this and additional works at: https://scholarworks.umt.edu/etd Let us know how access to this document benefits ou.y Recommended Citation Schulze, Judith Ann, "The toxigenic and hemolytic activity of Clostridium perfringens isolated from foods feces and soil" (1968). Graduate Student Theses, Dissertations, & Professional Papers. 6864. https://scholarworks.umt.edu/etd/6864 This Thesis is brought to you for free and open access by the Graduate School at ScholarWorks at University of Montana. It has been accepted for inclusion in Graduate Student Theses, Dissertations, & Professional Papers by an authorized administrator of ScholarWorks at University of Montana. For more information, please contact [email protected]. THE TOXIGENIC AND HEMOLYTIC ACTIVITY OF CLOSTRIDIUM PERFRINGMS ISOLATED FROM FOODS, FECES, AND SOIL By Judith Ao Schulze Bo Ao, Valparaiso University, 1965 Presented in partial fulfillment of the requirements for the degree of Master of Science UNIVERSITY OF M)NTANA 1968 Approved by; Chairman, Board of Examiners Dean,/waduate School ex'' May 27, 1968 Date Reproduced with permission of the copyright owner. Further reproduction prohibited without permission. UMI Number: EP37665 All rights reserved INFORMATION TO ALL USERS The quality of this reproduction is dependent upon the quality of the copy submitted. In the unlikely event that the author did not send a complete manuscript and there are missing pages, these will be noted.