Alm Ku 0099M 14701 DATA 1.Pdf
Total Page:16
File Type:pdf, Size:1020Kb
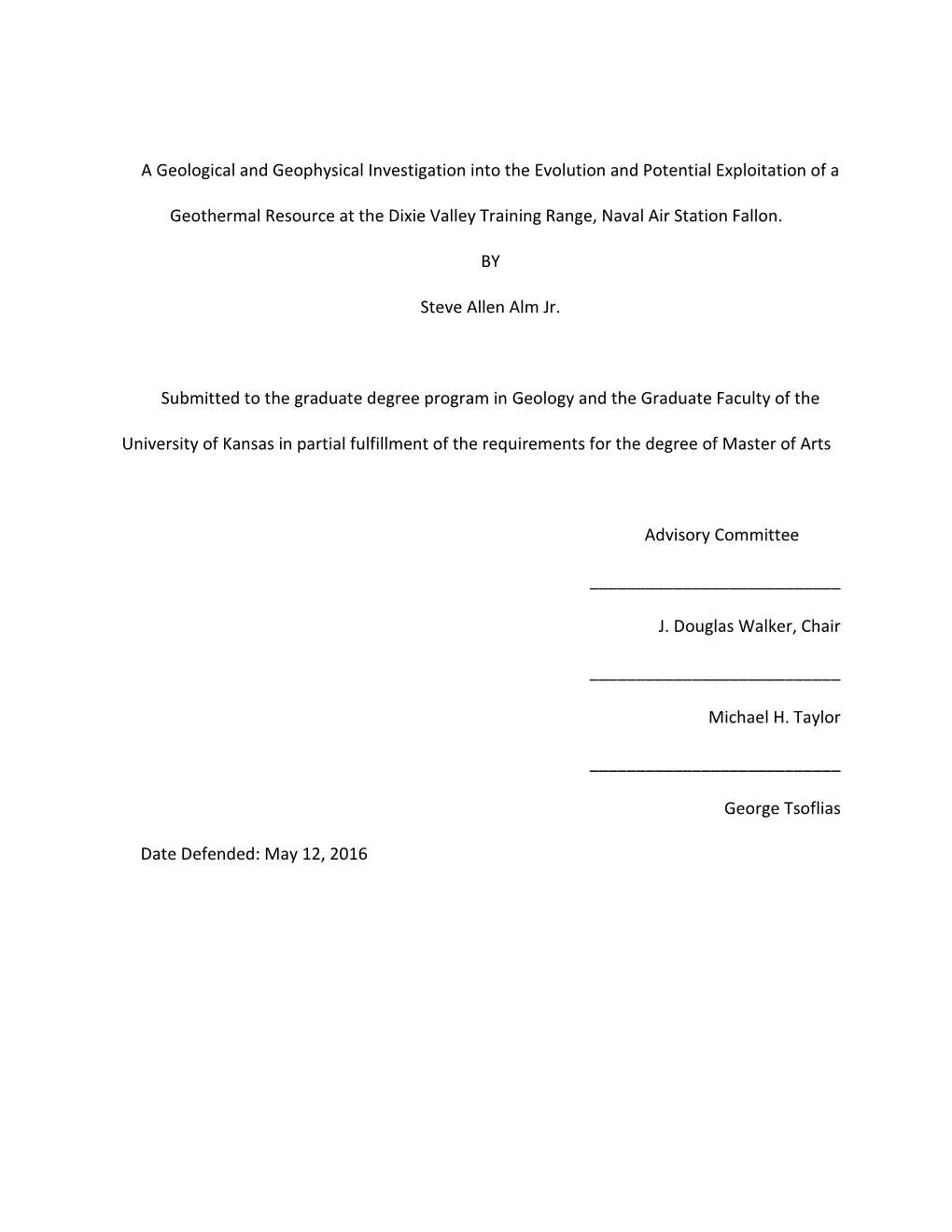
Load more
Recommended publications
-
Hadrian and the Greek East
HADRIAN AND THE GREEK EAST: IMPERIAL POLICY AND COMMUNICATION DISSERTATION Presented in Partial Fulfillment of the Requirements for the Degree Doctor of Philosophy in the Graduate School of the Ohio State University By Demetrios Kritsotakis, B.A, M.A. * * * * * The Ohio State University 2008 Dissertation Committee: Approved by Professor Fritz Graf, Adviser Professor Tom Hawkins ____________________________ Professor Anthony Kaldellis Adviser Greek and Latin Graduate Program Copyright by Demetrios Kritsotakis 2008 ABSTRACT The Roman Emperor Hadrian pursued a policy of unification of the vast Empire. After his accession, he abandoned the expansionist policy of his predecessor Trajan and focused on securing the frontiers of the empire and on maintaining its stability. Of the utmost importance was the further integration and participation in his program of the peoples of the Greek East, especially of the Greek mainland and Asia Minor. Hadrian now invited them to become active members of the empire. By his lengthy travels and benefactions to the people of the region and by the creation of the Panhellenion, Hadrian attempted to create a second center of the Empire. Rome, in the West, was the first center; now a second one, in the East, would draw together the Greek people on both sides of the Aegean Sea. Thus he could accelerate the unification of the empire by focusing on its two most important elements, Romans and Greeks. Hadrian channeled his intentions in a number of ways, including the use of specific iconographical types on the coinage of his reign and religious language and themes in his interactions with the Greeks. In both cases it becomes evident that the Greeks not only understood his messages, but they also reacted in a positive way. -
Mineral Resources of the Clan Alpine Mountains Wilderness Study Area, Churchill County, Nevada
Mineral Resources of the Clan Alpine Mountains Wilderness Study Area, Churchill County, Nevada U.S. GEOLOGICAL SURVEY BULLETIN 1727-B 1- J Chapter B Mineral Resources of the Clan Alpine Mountains Wilderness Study Area, Churchill County, Nevada By RICHARD F. HARDYMAN, WILLIAM E. BROOKS, MICHAEL J. BLASKOWSKI, HARLAN N. BARTON, and DAVID A. PONCE U.S. Geological Survey JERRY E. OLSON U.S. Bureau of Mines U.S. GEOLOGICAL SURVEY BULLETIN 1727 MINERAL RESOURCES OF WILDERNESS STUDY AREAS WEST-CENTRAL NEVADA DEPARTMENT OF THE INTERIOR DONALD PAUL MODEL, Secretary U. S. GEOLOGICAL SURVEY Dallas L. Peck, Director UNITED STATES GOVERNMENT PRINTING OFFICE: 1988 For sale by the Books and Open-File Reports Section U.S. Geological Survey Federal Center Box 25425 Denver, CO 80225 Library of Congress Cataloging-in-Publication Data Mineral resources of the Clan Alpine Mountains Wilderness Study Area, Churchill County, Nevada. (Mineral resources of wilderness study areas west-central Nevada ; ch. B) (U.S. Geological Survey bulletin ; 1727-B) Bibliography: p. Supt. of Docs, no.: I 19.3:1727-6 1. Mines and mineral resources Nevada Clan Alpine Mountains Wilderness. 2. Clan Alpine Mountains Wilderness (Nev.) I. Hardyman, Richard F. II. Series. III. Series: U.S. Geological Survey bulletin ; 1727-B. QE75.B9 no. 1727-B 557.3 s [553'.09793'52] 87-600445 [TN24.N3] STUDIES RELATED TO WILDERNESS Bureau of Land Management Wilderness Study Areas The Federal Land Policy and Management Act (Public Law 94-579, October 21, 1976) requires the U.S. Geological Survey and the U.S. Bureau of Mines to conduct mineral surveys on certain areas to determine the mineral values, if any, that may be present. -
The Developmentof Early Imperial Dress from the Tetrachs to The
View metadata, citation and similar papers at core.ac.uk brought to you by CORE provided by University of Birmingham Research Archive, E-theses Repository University of Birmingham Research Archive e-theses repository This unpublished thesis/dissertation is copyright of the author and/or third parties. The intellectual property rights of the author or third parties in respect of this work are as defined by The Copyright Designs and Patents Act 1988 or as modified by any successor legislation. Any use made of information contained in this thesis/dissertation must be in accordance with that legislation and must be properly acknowledged. Further distribution or reproduction in any format is prohibited without the permission of the copyright holder. The Development of Early Imperial Dress from the Tetrarchs to the Herakleian Dynasty General Introduction The emperor, as head of state, was the most important and powerful individual in the land; his official portraits and to a lesser extent those of the empress were depicted throughout the realm. His image occurred most frequently on small items issued by government officials such as coins, market weights, seals, imperial standards, medallions displayed beside new consuls, and even on the inkwells of public officials. As a sign of their loyalty, his portrait sometimes appeared on the patches sown on his supporters’ garments, embossed on their shields and armour or even embellishing their jewelry. Among more expensive forms of art, the emperor’s portrait appeared in illuminated manuscripts, mosaics, and wall paintings such as murals and donor portraits. Several types of statues bore his likeness, including those worshiped as part of the imperial cult, examples erected by public 1 officials, and individual or family groupings placed in buildings, gardens and even harbours at the emperor’s personal expense. -
C:\A Projects\AAA IBLA Decs\061IBLA\L370-375.Wpd
CHURCHILL COUNTY BOARD OF COMMISSIONERS IBLA 81-539 Decided February 17, 1982 Appeal from a decision of the Nevada State Director, Bureau of Land Management, denying a protest of wilderness study area designations. 8500 (N-932.6). Affirmed. 1. Federal Land Policy and Management Act of 1976: Wilderness--Wilderness Act--Words and Phrases. "Roadless." H.R. Rep. No. 94-1163, 94th Cong., 2d Sess. 17 (1976), provides a definition of "roadless" adopted by the Bureau of Land Management in its Wilderness Inventory Handbook. The word "roadless" refers to the absence of roads which have been improved and maintained by mechanical means to insure relatively regular and continuous use. A way maintained solely by the passage of vehicles does not constitute a road. 2. Federal Land Policy and Management Act of 1976: Wilderness--Wilderness Act Sights and sounds outside a wilderness study area will be considered during the study phase of the wilderness review process absent a finding by BLM during the inventory phase that such impacts are adjacent to the unit and are so extremely imposing that they cannot be ignored, and and if not considered, reasonable application of inventory guidelines would be questioned. 3. Federal Land Policy and Management Act of 1976: Wilderness--Wilderness Act The requirement in section 2(c) of the Wilderness Act of 1964, 16 U.S.C. § 1131(c) (1976), that a wilderness possess, inter alia, outstanding opportunities for solitude or a primitive and unconfined type of 61 IBLA 370 IBLA 81-539 recreation is properly construed to require outstanding opportunities for either solitude or a primitive and unconfined type of recreation; both need not be present in an inventory unit to allow the unit to enter the study phase of the wilderness review process. -
DOE/ET /27010-2 MC Coy AREA, NEVADA Geothermal Reservoir
t -- DOE/ET /27010-2 MC COy AREA, NEVADA Geothermal Reservo ir Assessment Case History Northern Basin and Range ANNUAL REPORT January 1980 - 31 December 1980 H. D. PILKINGTON August 1981 WORK PERFORMED UNDER CONTRACT DE AC 08-79 ET 27010 At-1AX EXPLORATION, INC. 7100 West 44th Ave. Wheat Rid~~t CO 80033 r TABLE OF CONTENTS Page ABSTRACT INTRODUCTION 2 EXPLORATION HISTORY 4 Geological Studies 4 Geochemical Studies . 6 Geophys i ca 1 Studies. 10 Exploration Drilling 13 \. TAB LE OF ILLUSTRATIONS Page Figure 1. Location map for the McCoy geothermal prospect 3 Figure 2. Geologic map of the McCoy geothermal prospect, Nevada (after Adams, Moore and Struhsacker, 1980) 5 Figure 3 Geologic cross-sections through well 14-7 (top) and well 66-8 (bottom) after Pilkington, 1980. 7 Figure 4 Location map of drill holes used on geochemical study of drill cuttings. 9 Figure 5 Contour map of mercury in drill cuttings from 120-160 foot interval at McCoy (Pilkington, 1980) . 11 Figure 6 Gravity profile with automatic interpretation for density (top) compared wi th geologic cross-section (bottom) 12 Figure 7 Resistivity at 5km depth from 10 magnetotelluric inve·rsion (Te mode) and location of survey lines 14 and stations Figure 8 MT section (Te mode, 10 inversion) along line C-C' compared with geologic section (after Lange, 1980) . 15 Figure 9 Survey location map of the McCoy prospect, after Wilt etal, 1980 . 16 Figure 10 MT section (Te mode 10 inversion) along line A-A" compared with EM data, after Lange, 1980 17 Page Table I Chemical analyses of McCoy Mine well and Well 66-8 waters 8 ABSTRACT The McCoy geothermal prospect is located at the junction of the Augusta Mountains, Clan Alpine Mountains and the New Pass Range. -
GEOLOGY of the SOUTHERN PART of the SAND SPRINGS RANGE, CHURCHILL COUNTY, NEVADA by S. K. M. Abdullah a Thesis Submitted in Part
GEOLOGY OF THE SOUTHERN PART OF THE SAND SPRINGS RANGE, CHURCHILL COUNTY, NEVADA By S. K. M. Abdullah A Thesis Submitted in Partial Fulfillment of the Requirements for the Degree of Master of Science Mackay School of Mines University of Nevada January, 1966 a. Approved by M. ,r Thesis Director Approved by j^T- \ Department Head Approved by Graduate Dean CONTENTS Page LIST OF ILLUSTRATIONS........................................ vi ABSTRACT . ................................................ 1 INTRODUCTION ................................................ 2 Location and Accessibility ................................ 2 Scope and Method of Investigation.......................... 2 Previous Investigations ...................... 5 Acknowledgments ............................................ 5 GEOLOGIC SETTING ............................................ 7 GRANITIC ROCKS ................................... 8 Introduction .............................................. 8 The Pluton ................................................ 8 Porphyritic Quartz Monzonite ............................ Granodiorite ................................. 11- Contacts and Minor Structures ............................... 12 Origin ...................................... 12 A g e ......................................................... 12 The Dike Rocks .......... - ................................ 18 Aplite-Pegmatite Dikes ..................................... 18 Andesite Dikes ............................................. 1^ Rhyolite Dikes ............................................ -
Are Plutons Assembled Over Millions of Years by Amalgamation From
1996; Petford et al., 2000). If plutons are emplaced by bulk magmatic flow, then during emplacement, the magma must Are plutons assembled contain a melt fraction of at least 30–50 vol% (Vigneresse et al., 1996). At lower melt fractions, crystals in the melt are welded to their neighbors; thus, a low melt-fraction material over millions of years by probably is better regarded not as magma but as a solid with melt-filled pore spaces because bulk flow of such a material requires pervasive solid-state deformation. amalgamation from small The concept of plutons as large ascending molten blobs (Fig. 1) is widespread in geologic thought and commonly magma chambers? guides interpretation of field relations (e.g., Buddington, 1959; Miller et al., 1988; Clarke, 1992; Bateman, 1992; Miller and Paterson, 1999). A contrasting view is that diapiric as- Allen F. Glazner, Department of Geological Sciences, cent of magma is too slow and energetically inefficient to be CB#3315, University of North Carolina, Chapel Hill, North geologically important, and large magma bodies only form Carolina 27599, USA, [email protected] at the emplacement level where they are fed by dikes (e.g., John M. Bartley, Department of Geology and Geophysics, Clemens and Mawer, 1992; Petford et al., 2000). Several lines University of Utah, Salt Lake City, Utah 84112, USA of evidence indicate that, regardless of the ascent mechanism, Drew S. Coleman, Walt Gray*, and Ryan Z. Taylor*, at least some plutons were emplaced incrementally over time Department of Geological Sciences, CB#3315, University of spans an order of magnitude longer than the thermal lifetime North Carolina, Chapel Hill, North Carolina 27599, USA of a large magmatic mass (Coleman et al., 2004). -
ABSTRACT Sarcophagi in Context: Identifying the Missing Sarcophagus of Helena in the Mausoleum of Constantina Jackson Perry
ABSTRACT Sarcophagi in Context: Identifying the Missing Sarcophagus of Helena in the Mausoleum of Constantina Jackson Perry Director: Nathan T. Elkins, Ph.D The Mausoleum of Constantina and Helena in Rome once held two sarcophagi, but the second has never been properly identified. Using the decoration in the mausoleum and recent archaeological studies, this thesis identifies the probable design of the second sarcophagus. This reconstruction is confirmed by a fragment in the Istanbul Museum, which belonged to the lost sarcophagus. This is contrary to the current misattribution of the fragment to the sarcophagus of Constantine. This is only the third positively identified imperial sarcophagus recovered in Constantinople. This identification corrects misconceptions about both the design of the mausoleum and the history of the fragment itself. Using this identification, this thesis will also posit that an altar was originally placed in the mausoleum, a discovery central in correcting misconceptions about the 4th century imperial liturgy. Finally, it will posit that the decorative scheme of the mausoleum was not random, but was carefully thought out in connection to the imperial funerary liturgy itself. APPROVED BY DIRECTOR OF HONORS THESIS _____________________________________________ Dr. Nathan T. Elkins, Art Department APPROVED BY THE HONORS PROGRAM ____________________________________________ Dr. Andrew Wisely, Director DATE: _____________________ SARCOPHAGI IN CONTEXT: IDENTIFYING THE MISSING SARCOPHAGUS OF HELENA IN THE MAUSOLEUM OF -
Imperial Porphyry Sarcophagi in Constantinople Author(S): A
Imperial Porphyry Sarcophagi in Constantinople Author(s): A. A. Vasiliev Reviewed work(s): Source: Dumbarton Oaks Papers, Vol. 4 (1948), pp. 1+3-26 Published by: Dumbarton Oaks, Trustees for Harvard University Stable URL: http://www.jstor.org/stable/1291047 . Accessed: 15/02/2013 15:16 Your use of the JSTOR archive indicates your acceptance of the Terms & Conditions of Use, available at . http://www.jstor.org/page/info/about/policies/terms.jsp . JSTOR is a not-for-profit service that helps scholars, researchers, and students discover, use, and build upon a wide range of content in a trusted digital archive. We use information technology and tools to increase productivity and facilitate new forms of scholarship. For more information about JSTOR, please contact [email protected]. Dumbarton Oaks, Trustees for Harvard University is collaborating with JSTOR to digitize, preserve and extend access to Dumbarton Oaks Papers. http://www.jstor.org This content downloaded on Fri, 15 Feb 2013 15:17:08 PM All use subject to JSTOR Terms and Conditions IMPERIAL PORPHYRY SARCOPHAGI IN CONSTANTINOPLE A. A. VASILIEV This content downloaded on Fri, 15 Feb 2013 15:17:08 PM All use subject to JSTOR Terms and Conditions D ESCRIPTIONSof marble sarcophagias well as sarcophagithem- selves have come down to us in both Byzantine literary sources and archaeological evidence of mediaeval Constantinople. Our infor- mation deals generally with the sarcophagi of the emperors, empresses, members of the imperial family, some patriarchs, and some very prominent laymen. These sarcophagi were made of various sorts of marble which dur- ing the Byzantine period were found in the vast territory of the Empire. -
F I N a L Mineral Assessment Report
BLM F I N A L MINERAL ASSESSMENT REPORT Battle Mountain District Office - Nevada J A N U A R Y 2 0 1 2 This page intentionally left blank Bureau of Land Management Mineral Assessment Report SUMMARY The Bureau of Land Management (BLM) Battle Mountain District Office (BMDO) is in the process of revising the district’s Resource Management Plan (RMP). As part of the RMP revision process, the BLM is required to prepare a Mineral Assessment Report providing information regarding mineral occurrences and potential within the BMDO Planning Area (planning area). This report provides an intermediate level of detail for mineral assessment as prescribed in BLM Manual 3060 (BLM 1994). Information presented in this report will be summarized and incorporated into an Environmental Impact Statement (EIS) for the proposed RMP and into the final RMP. The geologic history of central and southern Nevada and the planning area is very complex and includes two major cycles of sedimentation (western and eastern facies sources), episodic thrust faulting, mountain building, and associated intrusive and igneous activity. More recent geologic history includes a period of crustal extension that was accompanied by bimodal (rhyolite-basalt) volcanism, large volume caldera volcanism, and basin and range block-faulting resulting in high-levels of shallow crustal heat flow. The regional and local geologic setting has been instrumental in the location of and potential for numerous economic metallic mineral deposits in the planning area, as well as development of economic geothermal resources. MINING AND MINERAL ACTIVITY IN NEVADA Mineral exploration, particularly for gold, is an ongoing enterprise in Nevada by both operators of existing mines and by other exploration companies. -
Seeing in Stone
Seeing in Stone: Recycled Treasures in the Middle Ages By William John Burney Paton Master of Philosophy Faculty of Arts University of Glasgow Christie’s Education London Master’s Programme Early European Art September 2000 © William John Burney Paton ProQuest Number: 13818869 All rights reserved INFORMATION TO ALL USERS The quality of this reproduction is dependent upon the quality of the copy submitted. In the unlikely event that the author did not send a com plete manuscript and there are missing pages, these will be noted. Also, if material had to be removed, a note will indicate the deletion. uest ProQuest 13818869 Published by ProQuest LLC(2018). Copyright of the Dissertation is held by the Author. All rights reserved. This work is protected against unauthorized copying under Title 17, United States C ode Microform Edition © ProQuest LLC. ProQuest LLC. 789 East Eisenhower Parkway P.O. Box 1346 Ann Arbor, Ml 48106- 1346 Abstract The use of hardstones with various properties and histories in church objects was an iconographic statement in its own right. This iconography was shaped and manipulated through the new form or use of the object, usually through its new mounts. The pieces discussed contain layers and layers of meaning: religious resonances fitting for liturgical vessels or the political self-presentation of various patrons or institutions, exemplified by Roger II, Frederick II and Suger. Sometimes in investigating the core object conflicts seem apparent, like the use of Islamic vessels in the Church; sometimes a hardstone’s provenance could be its most valued feature, other times mounts were designed to mislead the viewer, manipulating the meaning of an object, disguising its origin for iconographic purposes. -
UNIVERSITY of NEVADA-RENO Nevada Bureau of Mines and Geology Un~Vrrsiryof Nevada-8.Eno Reno, Nevada 89557-0088 (702) 784-6691 FAX: (7G2j 784-1709
UNIVERSITY OF NEVADA-RENO Nevada Bureau of Mines and Geology Un~vrrsiryof Nevada-8.eno Reno, Nevada 89557-0088 (702) 784-6691 FAX: (7G2j 784-1709 NBMG OPEN-FILE REPORT 90-1 MINERAL RESOURCE INVENTORY BUREAU OF LAND MANAGEMENT, CARSON CITY DISTRICT, NEVADA Joseph V. Tingley This information should be considered preliminary. It has not been edited or checked for completeness or accuracy. Mineral Resource Inventory Bureau of Land Management, Carson City District, Nevada Prepared by: Joseph V. Tingley Prepared for: UNITED STATES DEPARTMENT OF E INTERIOR '\\ !\ BUREAU OF LAND MANAGEMENT Carson City Office Carson City, Nevada Under Cooperative Agreement 14-08-0001-A-0586 with the U.S. GEOLOGICAL SURVEY NEVADA BUREAU OF MINES AND GEOLOGY UNIVERSITY OF NEVADA, RENO January 1990 TABLE OF CONTENTS INTRODUCTION ........................ 3 LOCATION .......................... 4 MINERAL RESOURCES ...................... 4 MINING DISTRICTS AND AREAS .................. 6 ALLEN HOT SPRINGS AREA ................. 6 ALPINE DISTRICT .................... 7 AURORA DISTRICT .................... 10 BELL DISTRICT ..................... 13 BELLMOUNTAIN DISTRICT ................. 16 BENWAY DISTRICT .................... 19 BERNICE DISTRICT .................... 21 BOVARDDISTRICT .............23 BROKENHILLS DISTRICT ................. 27 BRUNERDISTRICT .................. 30 BUCKLEYDISTRICT ................. 32 BUCKSKINDISTRICT ............... 35 CALICO HILLS AREA ................... 39 CANDELARIA DISTRICT ................. 41 CARSON CITY DISTRICT .................. 44