JCU 55730-Malara-2017-Thesis.Pdf
Total Page:16
File Type:pdf, Size:1020Kb
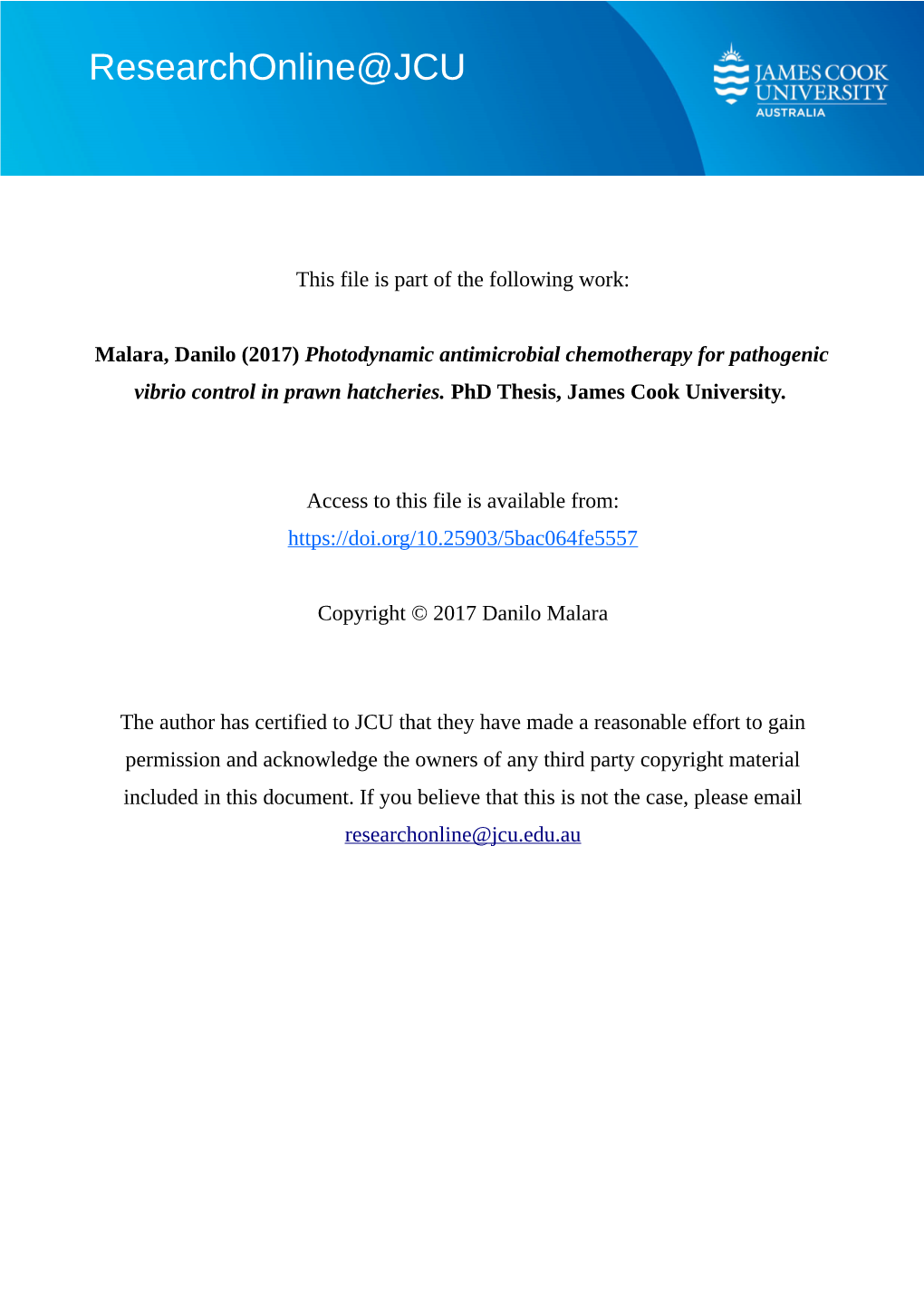
Load more
Recommended publications
-
Characterization and Complete Genome Sequence of Bacteriophage Vb Vc Srvc2, a Marine Phage That Infects Vibrio Campbellii
Characterization and complete genome sequence of bacteriophage vB_Vc_SrVc2, a marine phage that infects Vibrio campbellii Carlos Omar Lomelí-Ortega Centro de Investigaciónes Biológicas del Noroeste: Centro de Investigaciones Biologicas del Noroeste SC Alexis de Jesús Martínez-Sández Universidad Autónoma de Baja California Sur: Universidad Autonoma de Baja California Sur Diana Barajas-Sandoval Centro de Investigaciónes Biológicas del Noroeste: Centro de Investigaciones Biologicas del Noroeste SC Francisco Javier Magallón-Barajas Centro de Investigaciónes Biológicas del Noroeste: Centro de Investigaciones Biologicas del Noroeste SC Andrew Millard University of Leicester Juan Manuel Martínez-Villalobos Universidad Autónoma de Nuevo León: Universidad Autonoma de Nuevo Leon Elva Teresa Arechiga-Carvajal Universidad Autonoma de Nuevo Leon Eduardo Quiroz-Guzman ( [email protected] ) Centro de Investigaciones Biologicas del Noroeste SC https://orcid.org/0000-0002-4776-4564 Research Article Keywords: Vibrio campbellii, pathogen, bacteriophage, phage therapy Posted Date: August 20th, 2021 DOI: https://doi.org/10.21203/rs.3.rs-779229/v1 License: This work is licensed under a Creative Commons Attribution 4.0 International License. Read Full License Page 1/22 Abstract Vibrio campbellii is widely distributed in the marine environment and is an important pathogen of aquatic organisms such as shrimp, sh, and mollusks. The emergence of multi-drug resistance among these bacteria resulted in a worldwide public health problem, which requires alternative treatment approaches such as phage therapy. In the present study, we isolated a phage vB_Vc_SrVc2 from white shrimp hepatopancreas with symptoms of AHPND. Phage vB_Vc_SrVc2 is a member of the genus Maculvirus and the family Autographiviridae, with high lytic ability against Vibrio isolates. -
Delineating Virulence of Vibrio Campbellii
www.nature.com/scientificreports OPEN Delineating virulence of Vibrio campbellii: a predominant luminescent bacterial pathogen in Indian shrimp hatcheries Sujeet Kumar1*, Chandra Bhushan Kumar1,2, Vidya Rajendran1, Nishawlini Abishaw1, P. S. Shyne Anand1, S. Kannapan1, Viswas K. Nagaleekar3, K. K. Vijayan1 & S. V. Alavandi1 Luminescent vibriosis is a major bacterial disease in shrimp hatcheries and causes up to 100% mortality in larval stages of penaeid shrimps. We investigated the virulence factors and genetic identity of 29 luminescent Vibrio isolates from Indian shrimp hatcheries and farms, which were earlier presumed as Vibrio harveyi. Haemolysin gene-based species-specifc multiplex PCR and phylogenetic analysis of rpoD and toxR identifed all the isolates as V. campbellii. The gene-specifc PCR revealed the presence of virulence markers involved in quorum sensing (luxM, luxS, cqsA), motility (faA, lafA), toxin (hly, chiA, serine protease, metalloprotease), and virulence regulators (toxR, luxR) in all the isolates. The deduced amino acid sequence analysis of virulence regulator ToxR suggested four variants, namely A123Q150 (AQ; 18.9%), P123Q150 (PQ; 54.1%), A123P150 (AP; 21.6%), and P123P150 (PP; 5.4% isolates) based on amino acid at 123rd (proline or alanine) and 150th (glutamine or proline) positions. A signifcantly higher level of the quorum-sensing signal, autoinducer-2 (AI-2, p = 2.2e−12), and signifcantly reduced protease activity (p = 1.6e−07) were recorded in AP variant, whereas an inverse trend was noticed in the Q150 variants AQ and PQ. The pathogenicity study in Penaeus (Litopenaeus) vannamei juveniles revealed that all the isolates of AQ were highly pathogenic with Cox proportional hazard ratio 15.1 to 32.4 compared to P150 variants; PP (5.4 to 6.3) or AP (7.3 to 14). -
Siderophores from Marine Bacteria with Special Emphasis on Vibrionaceae
Archana et al Int. J. Pure App. Biosci. 7 (3): 58-66 (2019) ISSN: 2320 – 7051 Available online at www.ijpab.com DOI: http://dx.doi.org/10.18782/2320-7051.7492 ISSN: 2320 – 7051 Int. J. Pure App. Biosci. 7 (3): 58-66 (2019) Review Article Siderophores from Marine Bacteria with Special Emphasis on Vibrionaceae Archana V.1, K. Revathi2*, V. P. Limna Mol3, R. Kirubagaran3 1Department of Advanced Zoology and Biotechnology, Madras University, Chennai- 600005 2MAHER University, Chennai, Tamil Nadu – 600078 3Ocean Science and Technology for Islands, National Institute of Ocean Technology (NIOT), Ministry of Earth Sciences, Government of India, Pallikaranai, Chennai- 600100 *Corresponding Author E-mail: [email protected] Received: 11.04.2019 | Revised: 18.05.2019 | Accepted: 25.05.2019 ABSTRACT More than 500 siderophores have been isolated from a huge number of marine bacteria till date. With mankind’s ever-increasing search for novel molecules towards industrial and medical applications, siderophores have gained high importance. These chelating ligands have immense potential in promoting plant growth, drug-delivery, treatment of iron-overload, etc. Many of the potential siderophores have been isolated from bacteria like Pseudomonas, Bacillus, Nocardia, etc. Bacteria belonging to the family Vibrionaceae have recently gained focus owing to their rich potential in secreting siderophores. Many of the vibrionales, viz. Vibrio harveyii, V. anguillarium, V. campbellii. etc. are aquatic pathogens. These bacteria require iron for their growth and virulence, and hence produce a wide variety of siderophores. The genetic basis of siderophore production by Vibrio sp. has also been largely studied. Further detailed genetic analysis of the mode of siderophore production by Vibrionaceae would be highly effective to treat aquaculture diseases caused by these pathogenic organisms. -
A Novel Vibriophage Vb Vcas HC Containing Lysogeny-Related Gene Has Strong Lytic Ability Against Pathogenic Bacteria
Virologica Sinica www.virosin.org https://doi.org/10.1007/s12250-020-00271-w www.springer.com/12250 (0123456789().,-volV)(0123456789().,-volV) RESEARCH ARTICLE A Novel Vibriophage vB_VcaS_HC Containing Lysogeny-Related Gene Has Strong Lytic Ability against Pathogenic Bacteria 1,2 1,2 1,2 1 3 3 Chengcheng Li • Zengmeng Wang • Jiulong Zhao • Long Wang • Guosi Xie • Jie Huang • Yongyu Zhang1,2 Received: 2 March 2020 / Accepted: 8 June 2020 Ó Wuhan Institute of Virology, CAS 2020 Abstract To avoid the negative effects of antibiotics, using phage to prevent animal disease becomes a promising method in aquaculture. Here, a lytic phage provisionally named vB_VcaS_HC that can infect the pathogen (i.e., Vibrio campbellii 18) of prawn was isolated. The phage has an isometric head and a non-contractile tail. During phage infection, the induced host mortality in 5.5 h reached ca. 96%, with a latent period of 1.5 h and a burst size of 172 PFU/cell. It has an 81,566 bp circular dsDNA genome containing 121 open reading frames (ORFs), and ca. 71% of the ORFs are functionally unknown. Comparative genomic and phylogenetic analysis revealed that it is a novel phage belonging to Delepquintavirus, Siphoviridae, Caudovirales. In the phage genome, besides the ordinary genes related to structure assembly and DNA metabolism, there are 10 auxiliary metabolic genes. For the first time, the pyruvate phosphate dikinase (PPDK) gene was found in phages whose product is a key rate-limiting enzyme involving Embden-Meyerhof-Parnas (EMP) reaction. Interestingly, although the phage has a strong bactericidal activity and contains a potential lysogeny related gene, i.e., the recombinase (RecA) gene, we did not find the phage turned into a lysogenic state. -
Induction and Genome Analysis of HY01, a Newly Reported Prophage from an Emerging Shrimp Pathogen Vibrio Campbellii
microorganisms Article Induction and Genome Analysis of HY01, a Newly Reported Prophage from an Emerging Shrimp Pathogen Vibrio campbellii Taiyeebah Nuidate 1 , Aphiwat Kuaphiriyakul 1, Komwit Surachat 2,3 and Pimonsri Mittraparp-arthorn 1,3,* 1 Division of Biological Science, Faculty of Science, Prince of Songkla University, Hat Yai, Songkhla 90110, Thailand; [email protected] (T.N.); [email protected] (A.K.) 2 Division of Computational Science, Faculty of Science, Prince of Songkla University, Hat Yai, Songkhla 90110, Thailand; [email protected] 3 Molecular Evolution and Computational Biology Research Unit, Faculty of Science, Prince of Songkla University, Hat Yai, Songkhla 90110, Thailand * Correspondence: [email protected]; Tel.: +66-74-288-314 Abstract: Vibrio campbellii is an emerging aquaculture pathogen that causes luminous vibriosis in farmed shrimp. Although prophages in various aquaculture pathogens have been widely reported, there is still limited knowledge regarding prophages in the genome of pathogenic V. campbellii. Here, we describe the full-genome sequence of a prophage named HY01, induced from the emerging shrimp pathogen V. campbellii HY01. The phage HY01 was induced by mitomycin C and was morphologically characterized as long tailed phage. V. campbellii phage HY01 is composed of 41,772 bp of dsDNA with a G+C content of 47.45%. A total of 60 open reading frames (ORFs) were identified, of which 31 could be predicted for their biological functions. Twenty seven out of 31 predicted protein coding regions were matched with several encoded proteins of various Enterobacteriaceae, Pseudomonadaceae, Vibrionaceae, and other phages of Gram-negative bacteria. Interestingly, the comparative genome analysis revealed that the phage HY01 was only distantly related to Vibrio phage Va_PF430-3_p42 of Citation: Nuidate, T.; Kuaphiriyakul, fish pathogen V. -
Disease of Aquatic Organisms 103:133
Vol. 103: 133–139, 2013 DISEASES OF AQUATIC ORGANISMS Published March 26 doi: 10.3354/dao02572 Dis Aquat Org Identification and characterization of Vibrio harveyi associated with diseased abalone Haliotis diversicolor Qingru Jiang**, Liuyang Shi**, Caihuan Ke, Weiwei You, Jing Zhao* College of Ocean and Earth Science of Xiamen University, Xiamen 361005, China ABSTRACT: Mass mortality of farmed small abalone Haliotis diversicolor occurred in Fujian, China, from 2009 to 2011. Among isolates obtained from moribund abalones, the dominant species AP37 exhibited the strongest virulence. After immersion challenge with 106 CFU ml−1 of AP37, abalone mortalities of 0, 53 and 67% were induced at water temperatures of 20°C, 24°C, and 28°C, respectively. Following intramuscular injection, AP37 showed a low LD50 (median lethal concen- tration) value of 2.9 × 102 CFU g−1 (colony forming units per gram abalone wet body weight). The 6 −1 5 LT50 (median lethal time) values were 5.2 h for 1 × 10 CFU abalone , 8.4 h for 1 × 10 CFU abalone−1, and 21.5 h for 1 × 104 CFU abalone−1. For further analysis of virulence, AP37 was screened for the production of extracellular factors. The results showed that various factors includ- ing presence of flagella and production of extracellular enzymes, such as lipase, phospholipase and haemolysin, could be responsible for pathogenesis. Based on its 16S rRNA gene sequence, strain AP37 showed >98.8% similarity to Vibrio harveyi, V. campbellii, V. parahaemolyticus, V. algi nolyticus, V. na trie gens and V. rotiferianus, so it could not be identified by this method. -
Aquatic Microbial Ecology 80:15
The following supplement accompanies the article Isolates as models to study bacterial ecophysiology and biogeochemistry Åke Hagström*, Farooq Azam, Carlo Berg, Ulla Li Zweifel *Corresponding author: [email protected] Aquatic Microbial Ecology 80: 15–27 (2017) Supplementary Materials & Methods The bacteria characterized in this study were collected from sites at three different sea areas; the Northern Baltic Sea (63°30’N, 19°48’E), Northwest Mediterranean Sea (43°41'N, 7°19'E) and Southern California Bight (32°53'N, 117°15'W). Seawater was spread onto Zobell agar plates or marine agar plates (DIFCO) and incubated at in situ temperature. Colonies were picked and plate- purified before being frozen in liquid medium with 20% glycerol. The collection represents aerobic heterotrophic bacteria from pelagic waters. Bacteria were grown in media according to their physiological needs of salinity. Isolates from the Baltic Sea were grown on Zobell media (ZoBELL, 1941) (800 ml filtered seawater from the Baltic, 200 ml Milli-Q water, 5g Bacto-peptone, 1g Bacto-yeast extract). Isolates from the Mediterranean Sea and the Southern California Bight were grown on marine agar or marine broth (DIFCO laboratories). The optimal temperature for growth was determined by growing each isolate in 4ml of appropriate media at 5, 10, 15, 20, 25, 30, 35, 40, 45 and 50o C with gentle shaking. Growth was measured by an increase in absorbance at 550nm. Statistical analyses The influence of temperature, geographical origin and taxonomic affiliation on growth rates was assessed by a two-way analysis of variance (ANOVA) in R (http://www.r-project.org/) and the “car” package. -
Vibrio Parahaemolyticus Host Pathogen
FUNCTIONAL ANALYSIS OF THE ROLE OF ALTERNATIVE SIGMA FACTORS IN VIBRIO PARAHAEMOLYTICUS HOST PATHOGEN INTERACTIONS by Brandy L. Haines-Menges A dissertation submitted to the Faculty of the University of Delaware in partial fulfillment of the requirements for the degree of Doctor of Philosophy in Biological Sciences Summer 2015 © 2015 Brandy Haines-Menges All Rights Reserved ProQuest Number: 3730237 All rights reserved INFORMATION TO ALL USERS The quality of this reproduction is dependent upon the quality of the copy submitted. In the unlikely event that the author did not send a complete manuscript and there are missing pages, these will be noted. Also, if material had to be removed, a note will indicate the deletion. ProQuest 3730237 Published by ProQuest LLC (2015). Copyright of the Dissertation is held by the Author. All rights reserved. This work is protected against unauthorized copying under Title 17, United States Code Microform Edition © ProQuest LLC. ProQuest LLC. 789 East Eisenhower Parkway P.O. Box 1346 Ann Arbor, MI 48106 - 1346 FUNCTIONAL ANALYSIS OF THE ROLE OF ALTERNATIVE SIGMA FACTORS IN VIBRIO PARAHAEMOLYTICUS HOST PATHOGEN INTERACTIONS by Brandy L. Haines-Menges Approved: __________________________________________________________ Robin W. Morgan, Ph.D. Chair of the Department of Biological Sciences Approved: __________________________________________________________ George H. Watson, Ph.D. Dean of the College of Arts and Sciences Approved: __________________________________________________________ James G. Richards, Ph.D. Vice Provost for Graduate and Professional Education I certify that I have read this dissertation and that in my opinion it meets the academic and professional standard required by the University as a dissertation for the degree of Doctor of Philosophy. -
Identification and Initial Characterization of Prophages in Vibrio Campbellii
RESEARCH ARTICLE Identification and Initial Characterization of Prophages in Vibrio campbellii Nicola Lorenz1, Matthias Reiger1¤a, Mauricio Toro-Nahuelpan2,3, Andreas Brachmann4, Lisa Poettinger1, Laure Plener1¤b, Jürgen Lassak1, Kirsten Jung1* 1 Munich Center for Integrated Protein Science (CiPSM) at the Department of Biology I, Microbiology, Ludwig-Maximilians-Universität München, Martinsried, Germany, 2 Department of Microbiology, University of Bayreuth, Bayreuth, Germany, 3 Department of Molecular Structural Biology, Max-Planck-Institute of Biochemistry, Martinsried, Germany, 4 Department of Biology I, Genetics, Ludwig-Maximilians-Universität München, Martinsried, Germany ¤a Current address: Chair and Institute of Environmental Medicine, UNIKA-T, Technical University of Munich and Helmholtz Zentrum München–German Research Center for Environmental Health, Augsburg, Germany ¤b Current address: Gene & GreenTK, Faculté de Médecine, Marseille, France a11111 * [email protected] Abstract Phages are bacteria targeting viruses and represent the most abundant biological entities on earth. Marine environments are exceptionally rich in bacteriophages, harboring a total of OPEN ACCESS 4x1030 viruses. Nevertheless, marine phages remain poorly characterized. Here we Citation: Lorenz N, Reiger M, Toro-Nahuelpan M, describe the identification of intact prophage sequences in the genome of the marine γ-pro- Brachmann A, Poettinger L, Plener L, et al. (2016) Identification and Initial Characterization of teobacterium Vibrio campbellii ATCC BAA-1116 (formerly known as V. harveyi ATCC BAA- Prophages in Vibrio campbellii. PLoS ONE 11(5): 1116), which presumably belong to the family of Myoviridae. One prophage was found on e0156010. doi:10.1371/journal.pone.0156010 chromosome I and shows significant similarities to the previously identified phage ΦHAP-1. Editor: Baochuan Lin, Naval Research Laboratory, The second prophage region is located on chromosome II and is related to Vibrio phage UNITED STATES kappa. -
A Case Report on the Survivability of Marine Luminous Bacteria Vibrio Campbellii STF1 Under Starvation Conditions
Research Article Oceanogr Fish Open Access J Volume 5 Issue 5 - January 2018 DOI: 10.19080/OFOAJ.2018.05.555671 Copyright © All rights are reserved by Ramesh CH A Case Report on the Survivability of Marine Luminous Bacteria Vibrio campbellii STF1 under Starvation Conditions Ramesh CH1,2* and Mohanraju R2 1Department of Ocean Studies and Marine Biology, Pondicherry University, Port Blair-744102, Andaman and Nicobar Islands, India 2Andaman and Nicobar Centre for Ocean Science and Technology, ESSO-NIOT, Dollygunj, Port Blair, Andaman and Nicobar Islands-744103, India Submission: November 28, 2017; Published: January 08, 2018 *Corresponding author: Ramesh CH, Andaman and Nicobar Centre for Ocean Science and Technology, ESSO-NIOT, Dollygunj, Port Blair, Andaman and Nicobar Islands-744103, India, Email: Abstract Recently luminous Vibrio campbellii Vibrio campbellii has been recognised as a cosmopolitanSynanceia pathogenverrucosa in seafood and aquaculture industry. The significance of this study is to present the findings derived from longer-term starvation experiment on intense luminescence producing bacteria strain STF1. This strain was isolated from surface swab of (stone fish) and subjected to the starvation-survival procedure. The viability rate of this culture was determined following spread plate method by counting viable cells in starter inoculum and in the same starvation culture. It was observed that 90 to 95% of luminous bacteria could remain viable for over 4 years under nutrient deficiency condition.Keywords: This Luminous study also bacteria; determines Vibrio thecampbellii; effectiveness Starvation; of the preservationSurvival of luminous bacteria in sterile sweater. Introduction Nutrients are one of the main factors that are necessary Synanceia verrucosa for the survival of any microorganism or macro organism. -
Function and Regulation of Vibrio Campbellii Proteorhodopsin: Acquired Phototrophy in a Classical Organoheterotroph
Function and Regulation of Vibrio campbellii Proteorhodopsin: Acquired Phototrophy in a Classical Organoheterotroph Zheng Wang1, Thomas J. O’Shaughnessy1, Carissa M. Soto1, Amir M. Rahbar2, Kelly L. Robertson1, Nikolai Lebedev1, Gary J. Vora1* 1 Center for Bio/Molecular Science and Engineering, Naval Research Laboratory, Washington, D.C., United States of America, 2 National Cancer Institute, Bethesda, Maryland, United States of America Abstract Proteorhodopsins (PRs) are retinal-binding photoproteins that mediate light-driven proton translocation across prokaryotic cell membranes. Despite their abundance, wide distribution and contribution to the bioenergy budget of the marine photic zone, an understanding of PR function and physiological significance in situ has been hampered as the vast majority of PRs studied to date are from unculturable bacteria or culturable species that lack the tools for genetic manipulation. In this study, we describe the presence and function of a horizontally acquired PR and retinal biosynthesis gene cluster in the culturable and genetically tractable bioluminescent marine bacterium Vibrio campbellii. Pigmentation analysis, absorption spectroscopy and photoinduction assays using a heterologous over-expression system established the V. campbellii PR as a functional green light absorbing proton pump. In situ analyses comparing PR expression and function in wild type (WT) V. campbellii with an isogenic DpR deletion mutant revealed a marked absence of PR membrane localization, pigmentation and light-induced proton pumping in the DpR mutant. Comparative photoinduction assays demonstrated the distinct upregulation of pR expression in the presence of light and PR-mediated photophosphorylation in WT cells that resulted in the enhancement of cellular survival during respiratory stress. In addition, we demonstrate that the master regulator of adaptive stress response and stationary phase, RpoS1, positively regulates pR expression and PR holoprotein pigmentation. -
Inhibitory Activity of Essential Oils Against Vibrio Campbellii and Vibrio Parahaemolyticus
microorganisms Article Inhibitory Activity of Essential Oils against Vibrio campbellii and Vibrio parahaemolyticus Xiaoting Zheng 1,2 , Adam F. Feyaerts 3,4 , Patrick Van Dijck 3,4 and Peter Bossier 1,* 1 Laboratory of Aquaculture & Artemia Reference Center, Department of Animal Production, Faculty of Bioscience Engineering, Ghent University, 9000 Ghent, Belgium; [email protected] 2 Key Laboratory of South China Sea Fishery Resources Exploitation & Utilization, Ministry of Agriculture and Rural Affairs, South China Sea Fisheries Research Institute, Chinese Academy of Fishery Sciences, Guangzhou 510300, China 3 VIB-KU Leuven Center for Microbiology, 3001 Leuven, Belgium; [email protected] (A.F.F.); [email protected] (P.V. D.) 4 Laboratory of Molecular Cell Biology, KU Leuven, 3001 Leuven, Belgium * Correspondence: [email protected] Received: 30 September 2020; Accepted: 4 December 2020; Published: 8 December 2020 Abstract: Vibriosis, caused by Vibrio strains, is an important bacterial disease and capable of causing significant high mortality in aquatic animals. Essential oils (EOs) have been considered as an alternative approach for the treatment of aquatic bacterial diseases. In this study, we evaluated the antibacterial activity of essential oils (n = 22) or essential oil components (EOCs, n = 12) against Vibrio strains belonging to the harveyi clade. It was verified by three different approaches, e.g., (i) a bacterial growth assay, comparing Vibrio growth with or without EO(C)s at various concentrations; (ii) a vapor-phase-mediated susceptibility assay, comparing the effect of EO(C)s on bacterial growth through the vapor phase; and (iii) a quorum sensing-inhibitory assay, based on specific inhibition of quorum sensing-regulated bioluminescence.