Information to Users
Total Page:16
File Type:pdf, Size:1020Kb
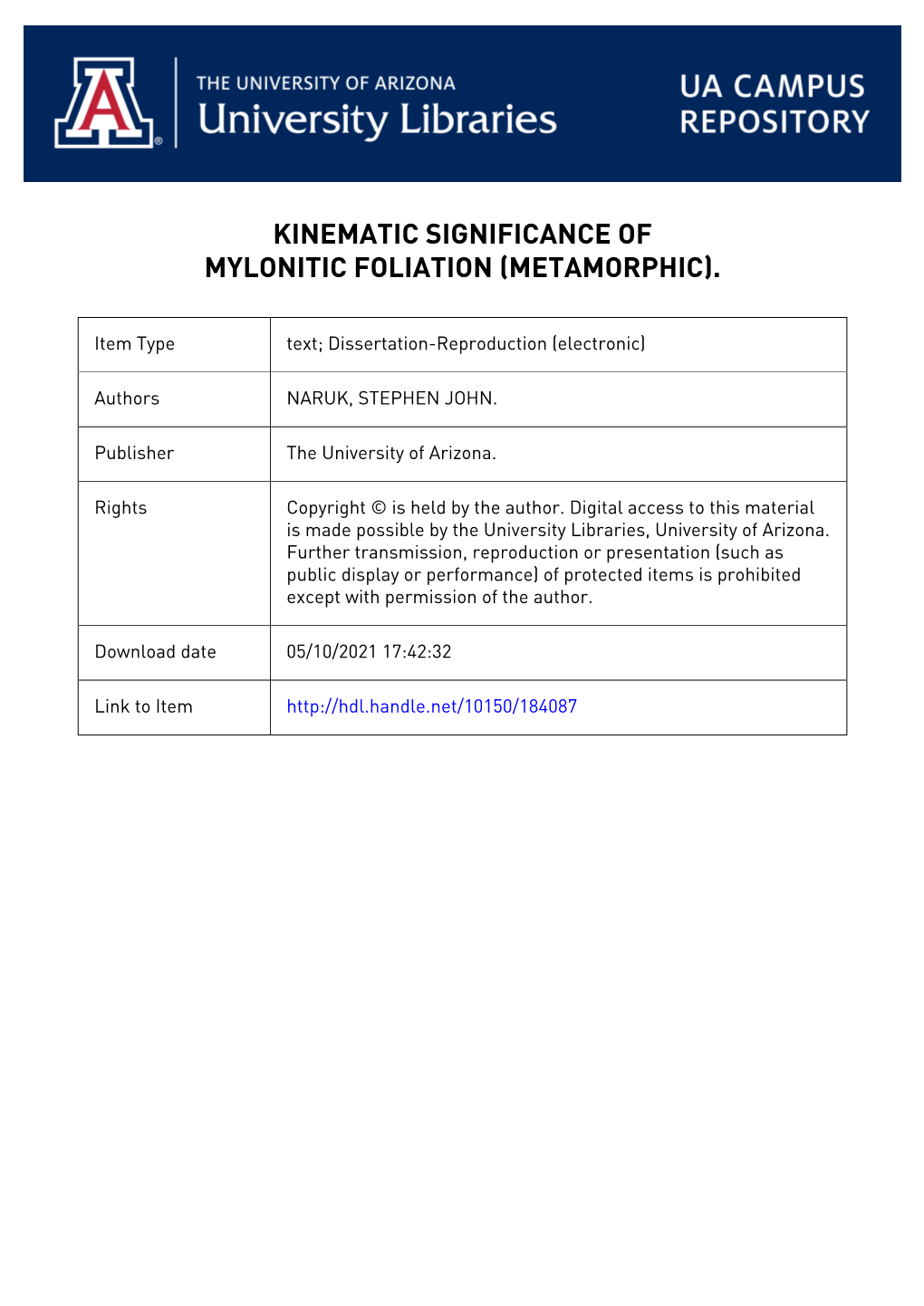
Load more
Recommended publications
-
Tectonic Imbrication and Foredeep Development in the Penokean
Tectonic Imbrication and Foredeep Development in the Penokean Orogen, East-Central Minnesota An Interpretation Based on Regional Geophysics and the Results of Test-Drilling The Penokean Orogeny in Minnesota and Upper Michigan A Comparison of Structural Geology U.S. GEOLOGICAL SURVEY BULLETIN 1904-C, D AVAILABILITY OF BOOKS AND MAPS OF THE U.S. GEOLOGICAL SURVEY Instructions on ordering publications of the U.S. Geological Survey, along with prices of the last offerings, are given in the cur rent-year issues of the monthly catalog "New Publications of the U.S. Geological Survey." Prices of available U.S. Geological Sur vey publications released prior to the current year are listed in the most recent annual "Price and Availability List." Publications that are listed in various U.S. Geological Survey catalogs (see back inside cover) but not listed in the most recent annual "Price and Availability List" are no longer available. Prices of reports released to the open files are given in the listing "U.S. Geological Survey Open-File Reports," updated month ly, which is for sale in microfiche from the U.S. Geological Survey, Books and Open-File Reports Section, Federal Center, Box 25425, Denver, CO 80225. Reports released through the NTIS may be obtained by writing to the National Technical Information Service, U.S. Department of Commerce, Springfield, VA 22161; please include NTIS report number with inquiry. Order U.S. Geological Survey publications by mail or over the counter from the offices given below. BY MAIL OVER THE COUNTER Books Books Professional Papers, Bulletins, Water-Supply Papers, Techniques of Water-Resources Investigations, Circulars, publications of general in Books of the U.S. -
Shear Zone-Related Folds
Journal of Structural Geology 27 (2005) 1229–1251 www.elsevier.com/locate/jsg Shear zone-related folds Jordi Carreras, Elena Druguet*, Albert Griera Departament de Geologia, Universitat Auto`noma de Barcelona, 08193 Bellaterra, Spain Received 18 April 2003; received in revised form 27 February 2004; accepted 14 June 2004 Available online 30 November 2004 Abstract Folds in ductile shear zones are common structures that have a variety of origins. These can be pre-existing folds that become modified or folds developed during the shearing event. Among the syn-shearing folds, a second subdivision is based on the relative age of the folded surface, which can be pre-existing or newly formed during the shearing event. In each of the three categories final fold geometry and orientation show complex relationships with the kinematic frame. The final fold geometry depends on the vorticity within the shear zone, the rheology and the initial orientation of the folded surface relative to the kinematic framework. It follows that folds are complex structures, difficult to use as kinematic indicators. However, in shear zones where undeformed wall rocks with pre-shear structures are accessible and where kinematics can be well established, folds can provide a valuable natural means to understand the initiation and evolution of structures under non-coaxial regimes. We point to the need of discriminating among different plausible categories, based on the nature of the folded surface and on the inherent structural features of each category. q 2004 Elsevier Ltd. All rights reserved. Keywords: Fold; Shear zone; Geometry; Kinematics; Cap de Creus 1. Introduction final geometry, symmetry and orientation of a shear-related fold are influenced by many variables other than the shear Folds are common structures in many ductile shear sense. -
Characterization of Olivine Fabrics and Mylonite in the Presence of Fluid
Jung et al. Earth, Planets and Space 2014, 66:46 http://www.earth-planets-space.com/content/66/1/46 FULL PAPER Open Access Characterization of olivine fabrics and mylonite in the presence of fluid and implications for seismic anisotropy and shear localization Sejin Jung1, Haemyeong Jung1* and Håkon Austrheim2 Abstract The Lindås Nappe, Bergen Arc, is located in western Norway and displays two high-grade metamorphic structures. A Precambrian granulite facies foliation is transected by Caledonian fluid-induced eclogite-facies shear zones and pseudotachylytes. To understand how a superimposed tectonic event may influence olivine fabric and change seismic anisotropy, two lenses of spinel lherzolite were studied by scanning electron microscope (SEM) and electron back-scattered diffraction (EBSD) techniques. The granulite foliation of the surrounding anorthosite complex is displayed in ultramafic lenses as a modal variation in olivine, pyroxenes, and spinel, and the Caledonian eclogite-facies structure in the surrounding anorthosite gabbro is represented by thin (<1 cm) garnet-bearing ultramylonite zones. The olivine fabrics in the spinel bearing assemblage were E-type and B-type and a combination of A- and B-type lattice preferred orientations (LPOs). There was a change in olivine fabric from a combination of A- and B-type LPOs in the spinel bearing assemblage to B- and E-type LPOs in the garnet lherzolite mylonite zones. Fourier transform infrared (FTIR) spectroscopy analyses reveal that the water content of olivine in mylonite is much higher (approximately 600 ppm H/Si) than that in spinel lherzolite (approximately 350 ppm H/Si), indicating that water caused the difference in olivine fabric. -
Anja SCHORN & Franz NEUBAUER
Austrian Journal of Earth Sciences Volume 104/2 22 - 46 Vienna 2011 Emplacement of an evaporitic mélange nappe in central Northern Calcareous Alps: evidence from the Moosegg klippe (Austria)_______________________________________________ Anja SCHORN*) & Franz NEUBAUER KEYWORDS thin-skinned tectonics deformation analysis Dept. Geography and Geology, University of Salzburg, Hellbrunnerstr. 34, A-5020 Salzburg, Austria; sulphate mélange fold-thrust belt *) Corresponding author, [email protected] mylonite Abstract For the reconstruction of Alpine tectonics, the Permian to Lower Triassic Haselgebirge Formation of the Northern Calcareous Alps (NCA) (Austria) plays a key role in: (1) understanding the origin of Haselgebirge bearing nappes, (2) revealing tectonic processes not preserved in other units, and (3) in deciphering the mode of emplacement, namely gravity-driven or tectonic. With these aims in mind, we studied the sulphatic Haselgebirge exposed to the east of Golling, particularly the gypsum quarry Moosegg and its surroun- dings located in the central NCA. There, overlying the Lower Cretaceous Rossfeld Formation, the Haselgebirge Formation forms a tectonic klippe (Grubach klippe) preserved in a synform, which is cut along its northern edge by the ENE-trending high-angle normal Grubach fault juxtaposing Haselgebirge to the Upper Jurassic Oberalm Formation. According to our new data, the Haselgebirge bearing nappe was transported over the Lower Cretaceous Rossfeld Formation, which includes many clasts derived from the Hasel- gebirge Fm. and its exotic blocks deposited in front of the incoming nappe. The main Haselgebirge body contains foliated, massive and brecciated anhydrite and gypsum. A high variety of sulphatic fabrics is preserved within the Moosegg quarry and dominant gyp- sum/anhydrite bodies are tectonically mixed with subordinate decimetre- to meter-sized tectonic lenses of dark dolomite, dark-grey, green and red shales, pelagic limestones and marls, and abundant plutonic and volcanic rocks as well as rare metamorphic rocks. -
Mylonite Zones in the Crystalline Basement Rocks of Sixmile Creek and Yankee Jim Canyon Park County Montana
University of Montana ScholarWorks at University of Montana Graduate Student Theses, Dissertations, & Professional Papers Graduate School 1982 Mylonite zones in the crystalline basement rocks of Sixmile Creek and Yankee Jim Canyon Park County Montana Robert Robert Burnham The University of Montana Follow this and additional works at: https://scholarworks.umt.edu/etd Let us know how access to this document benefits ou.y Recommended Citation Burnham, Robert Robert, "Mylonite zones in the crystalline basement rocks of Sixmile Creek and Yankee Jim Canyon Park County Montana" (1982). Graduate Student Theses, Dissertations, & Professional Papers. 4677. https://scholarworks.umt.edu/etd/4677 This Thesis is brought to you for free and open access by the Graduate School at ScholarWorks at University of Montana. It has been accepted for inclusion in Graduate Student Theses, Dissertations, & Professional Papers by an authorized administrator of ScholarWorks at University of Montana. For more information, please contact [email protected]. COPYRIGHT ACT OF 1976 This is an unpublished manuscript in which copyright sub s is t s . Any further reprinting of its contents must be approved BY THE AUTHOR. Mansfield Library U niversity of Montana Date: 19 8 2 MYLONITE ZONES IN THE CRYSTALLINE BASEMENT ROCKS OF SIXMILE CREEK AND YANKEE JIM CANYON, PARK COUNTY, MONTANA by Robert Burnham B.A., Dartmouth, 1980 Presented in partial fulfillment of the requirements for the degree of Master of Science UNIVERSITY OF MONTANA 1982 Approved by: UMI Number: EP40141 All rights reserved INFORMATION TO ALL USERS The quality of this reproduction is dependent upon the quality of the copy submitted. In the unlikely event that the author did not send a complete manuscript and there are missing pages, these will be noted. -
Metamorphic Fabrics
11/30/2015 Geol341 J. Toro Topics • Fabrics • Foliation, cleavage, lineation Metamorphic Rocks and – Cleavage and Folds –Geometry Cleavage Development – Strain significance • Origin of Cleavage – Pressure solution – Passive rotation – Recrystallization • Shear zones Many diagrams are from Earth Structure, van der Pluijm and Marshak, 2004 2013 Geothermal Gradient and Metamorphism Naming of Metamorphic Rocks Slatey cleavage Gneiss Segregation of mafic and felsic components Where does it come from? 1 11/30/2015 Looks like bedding, but is it? Metamorphic layering Brooks Range, AK Quartz-mica schist Isoclinal Fold Transposition of Layering Fabric “Arrangement of component features in a rock” van der Pluijm & Marshak •Includes: •Texture •Composition •Microstructure •Preferred Orientation Horizontal fabric => Vertical fabric 2 11/30/2015 Quartz-mica schist Fabric Elements •Bedding (S0) •Compositional layering •Crystallographic orientation •Fold Hinges •Cleavage planes (S1) •Mineral elongation lineation Passchier and Trouw (1996) Metamorphic Fabrics Metamorphic Fabrics • Foliation : Cleavage, Schistosity • Foliation • Lineation: Mineral Lineation, Intersection Lineation – Cleavage – Schistosity • Lineation Random fabric S-tectonite L-tectonite L/S-tectonite Foliation Lineation S-Tectonites L-Tectonites Schists Columbia Pluton, VA Lineated Gneiss USGS photo U. Western Ontario photo 3 11/30/2015 L-S Tectonites 3D Strain - Flinn diagram No strain along 3rd dimension Cigars S =S >S S1/S2 1 2 3 S1>S2=S3 Lineated and foliated gneiss, Himalayas Prolate -
Physical Properties of Surface Outcrop Cataclastic Fault Rocks, Alpine Fault, New Zealand
Article Volume 13, Number 1 28 January 2012 Q01018, doi:10.1029/2011GC003872 ISSN: 1525-2027 Physical properties of surface outcrop cataclastic fault rocks, Alpine Fault, New Zealand C. Boulton Department of Geological Sciences, University of Canterbury, PB 4800, Christchurch 8042, New Zealand ([email protected]) B. M. Carpenter Department of Geosciences, Pennsylvania State University, 522 Deike Building, University Park, Pennsylvania 16802, USA V. Toy Department of Geology, University of Otago, P.O. Box 56, Dunedin 9054, New Zealand C. Marone Department of Geosciences, Pennsylvania State University, 522 Deike Building, University Park, Pennsylvania 16802, USA [1] We present a unified analysis of physical properties of cataclastic fault rocks collected from surface exposures of the central Alpine Fault at Gaunt Creek and Waikukupa River, New Zealand. Friction experi- ments on fault gouge and intact samples of cataclasite were conducted at 30–33 MPa effective normal stress (sn′) using a double-direct shear configuration and controlled pore fluid pressure in a true triaxial pressure vessel. Samples from a scarp outcrop on the southwest bank of Gaunt Creek display (1) an increase in fault normal permeability (k=7.45 Â 10À20 m2 to k = 1.15 Â 10À16 m2), (2) a transition from frictionally weak (m = 0.44) fault gouge to frictionally strong (m = 0.50–0.55) cataclasite, (3) a change in friction rate depen- dence (a-b) from solely velocity strengthening, to velocity strengthening and weakening, and (4) an increase in the rate of frictional healing with increasing distance from the footwall fluvioglacial gravels contact. At Gaunt Creek, alteration of the primary clay minerals chlorite and illite/muscovite to smectite, kaolinite, and goethite accompanies an increase in friction coefficient (m = 0.31 to m = 0.44) and fault- perpendicular permeability (k=3.10 Â 10À20 m2 to k = 7.45 Â 10À20 m2). -
Bulletin of the Mineral Research and Exploration
BULLETIN OF THE MINERAL RESEARCH AND EXPLORATION Foreign Edition October 1985-April 1986 Number : 105/106 CONTENTS Determination of the sense of shear using the orientation of shear band foliation in mylonites: field evidence from the Keban complex, Eastern Turkey Gültekin Savcı 1 Biostratigraphy and paleontology of the Medik-Ebreme (NW Malatya) area Sefer Örçen 15 Geochemical prospecting for carbonate-bearing lead-zinc deposits in the Western Zamantı (Aladağlar-Yahyalı) region Ahmet Ayhan and Mehmet Erbayar 46 Remarks on different methods for analyzing trona and soda samples Gülay Ataman; Süheyla Tuncer and Nurgiin Güngör 57 Distribution of the major and trace-elements in the volcanic rocks of Yozgat area, Turkey Gönül Büyükönal 68 Geochronological data from the southern part (Niğde area) of the Central Anatolian massif M. Cemal Göncüoğlu 83 Determining two dimensions of a concealed chromite ore by microcomputer modelling of magnetic total field intensity profile Doğan Aydal 97 Lacazina oeztemueri Sirel 1981 renamed as Pseudolacazina oeztemueri (Sirel) from the Thanetian limestone (Central Turkey) Ercüment Sirel 123 Abstracts of the papers published only in the Turkish edition of this bulletin 127 Bu nüshada yazı işlerini fiilen idare edenler-Editors: İsmail SEYHAN-Özcan YAZLAK GENERAL DIRECTOR M. Sıtkı SANCAR EDITORIAL BOARD İsmail SEYHAN (President) Zeki DAĞER Tandoğan ENGİN M. Cemal GÖNCÜOĞLU İsmail HENDEN Burhan KORKMAZER Ali Rıza MİDİLLİ Okan TEKELİ Özcan YAZLAK Mehmet C. YILDIZ PUBLICATION MANAGER Mualla ERGUN SECRETARY Gülgün HASBAY POSTAL ADDRESS Maden Tetkik ve Arama Genel Müdürlüğü Redaksiyon Kurulu Sekreterliği Ankara-TURKEY The Bulletin of the Mineral Research and Exploration (MTA) is published twice yearly. Each issue appears in Turkish and foreign editions. -
Structural Geology of Parautochthonous and Allochthonous Terranes of the Penokean Orogeny in Upper Michigan Comparisons with Northern Appalachian Tectonics
Structural Geology of Parautochthonous and Allochthonous Terranes of the Penokean Orogeny in Upper Michigan Comparisons with Northern Appalachian Tectonics U.S. GEOLOGICAL SURVEY BULLETIN 1904-Q AVAILABILITY OF BOOKS AND MAPS OF THE U.S. GEOLOGICAL SURVEY Instructions on ordering publications of the U.S. Geological Survey, along with the last offerings, are given in the current-year issues of the monthly catalog "New Publications of the U.S. Geological Survey." Prices of available U.S. Geological Survey publications released prior to the current year are listed in the most recent annual "Price and Availability List." Publications that are listed in various U.S. Geological Survey catalogs (see back inside cover) but not listed in the most recent annual "Price and Availability List" are no longer available. Prices of reports released to the open files are given in the listing "U.S. Geological Survey Open-File Reports," updated monthly, which is for sale in microfiche from the U.S. Geological Survey, Book and Open-File Report Sales, Box 25286, Building 810, Denver Federal Center, Denver, CO 80225 Order U.S. Geological Survey publications by mail or over the counter from the offices given below. BY MAIL OVER THE COUNTER Books Books Professional Papers, Bulletins, Water-Supply Papers, Tech Books of the U.S. Geological Survey are available over the niques of Water-Resources Investigations, Circulars, publications counter at the following U.S. Geological Survey offices, all of of general interest (such as leaflets, pamphlets, booklets), single which are authorized agents of the Superintendent of Documents. copies of periodicals (Earthquakes & Volcanoes, Preliminary De termination of Epicenters), and some miscellaneous reports, includ ANCHORAGE, Alaska-Rm. -
Grand Canyon
U.S. Department of the Interior Geologic Investigations Series I–2688 14 Version 1.0 4 U.S. Geological Survey 167.5 1 BIG SPRINGS CORRELATION OF MAP UNITS LIST OF MAP UNITS 4 Pt Ph Pamphlet accompanies map .5 Ph SURFICIAL DEPOSITS Pk SURFICIAL DEPOSITS SUPAI MONOCLINE Pk Qr Holocene Qr Colorado River gravel deposits (Holocene) Qsb FAULT CRAZY JUG Pt Qtg Qa Qt Ql Pk Pt Ph MONOCLINE MONOCLINE 18 QUATERNARY Geologic Map of the Pleistocene Qtg Terrace gravel deposits (Holocene and Pleistocene) Pc Pk Pe 103.5 14 Qa Alluvial deposits (Holocene and Pleistocene) Pt Pc VOLCANIC ROCKS 45.5 SINYALA Qti Qi TAPEATS FAULT 7 Qhp Qsp Qt Travertine deposits (Holocene and Pleistocene) Grand Canyon ၧ DE MOTTE FAULT Pc Qtp M u Pt Pleistocene QUATERNARY Pc Qp Pe Qtb Qhb Qsb Ql Landslide deposits (Holocene and Pleistocene) Qsb 1 Qhp Ph 7 BIG SPRINGS FAULT ′ × ′ 2 VOLCANIC DEPOSITS Dtb Pk PALEOZOIC SEDIMENTARY ROCKS 30 60 Quadrangle, Mr Pc 61 Quaternary basalts (Pleistocene) Unconformity Qsp 49 Pk 6 MUAV FAULT Qhb Pt Lower Tuckup Canyon Basalt (Pleistocene) ၣm TRIASSIC 12 Triassic Qsb Ph Pk Mr Qti Intrusive dikes Coconino and Mohave Counties, Pe 4.5 7 Unconformity 2 3 Pc Qtp Pyroclastic deposits Mr 0.5 1.5 Mၧu EAST KAIBAB MONOCLINE Pk 24.5 Ph 1 222 Qtb Basalt flow Northwestern Arizona FISHTAIL FAULT 1.5 Pt Unconformity Dtb Pc Basalt of Hancock Knolls (Pleistocene) Pe Pe Mၧu Mr Pc Pk Pk Pk NOBLE Pt Qhp Qhb 1 Mၧu Pyroclastic deposits Qhp 5 Pe Pt FAULT Pc Ms 12 Pc 12 10.5 Lower Qhb Basalt flows 1 9 1 0.5 PERMIAN By George H. -
Stylolites: a Review
Stylolites: a review Toussaint R.1,2,3*, Aharonov E.4, Koehn, D.5, Gratier, J.-P.6, Ebner, M.7, Baud, P.1, Rolland, A.1, and Renard, F.6,8 1Institut de Physique du Globe de Strasbourg, CNRS, University of Strasbourg, 5 rue Descartes, F- 67084 Strasbourg Cedex, France. Phone : +33 673142994. email : [email protected] 2 International Associate Laboratory D-FFRACT, Deformation, Flow and Fracture of Disordered Materials, France-Norway. 3SFF PoreLab, The Njord Centre, Department of Physics, University of Oslo, Norway. 4Institute of Earth Sciences, The Hebrew University, Jerusalem, 91904, Israel 5School of Geographical and Earth Sciences, University of Glasgow, UK 6University Grenoble Alpes, ISTerre, Univ. Savoie Mont Blanc, CNRS, IRD, IFSTTAR, 38000 Grenoble, France 7OMV Exploration & Production GmbH Trabrennstrasse 6-8, 1020 Vienna, Austria 8 The Njord Centre,PGP, Department of Geosciences, University of Oslo, Norway *corresponding author Highlights: . Stylolite formation depends on rock composition and structure, stress and fluids. Stylolite geometry, fractal and self-affine properties, network structure, are investigated. The experiments and physics-based numerical models for their formation are reviewed. Stylolites can be used as markers of strain, paleostress orientation and magnitude. Stylolites impact transport properties, as function of maturity and flow direction. Abstract Stylolites are ubiquitous geo-patterns observed in rocks in the upper crust, from geological reservoirs in sedimentary rocks to deformation zones, in folds, faults, and shear zones. These rough surfaces play a major role in the dissolution of rocks around stressed contacts, the transport of dissolved material and the precipitation in surrounding pores. Consequently, they 1 play an active role in the evolution of rock microstructures and rheological properties in the Earth’s crust. -
Albania): Oceanic Detachment Shear Zone
Research Paper GEOSPHERE Mylonites in ophiolite of Mirdita (Albania): Oceanic detachment shear zone 1 2 1 3 2 GEOSPHERE; v. 13, no. 1 A. Nicolas , A. Meshi , F. Boudier , D. Jousselin , and B. Muceku 1Geosciences, Université de Montpellier II, 34095 Montpellier, France 2Faculty of Geology, Polytechnic University of Tirana, Rruga Elbasanit, 1010, Albania doi:10.1130/GES01383.1 3Université de Lorraine, Ecole Nationale Supérieure Géologie Centre de Recherches Pétrographiques et Géochimiques (ENSG-CRPG), 54501 Vandoeuvre-les-Nancy, France 11 figures; 1 table ABSTRACT west (Fig. 1A). Based on several field campaigns in the northern part of Mirdita CORRESPONDENCE: Francoise .Boudier@ gm during the 1990s, it seems to be one of the few and, so far, the best ophio- .univ -montp2.fr The northern Mirdita ophiolite massifs in Albanian Dinarides formed at a lite where an oceanic core complex (OCC) has been identified, located within slow-spreading ridge, active during the Jurassic (160–165 Ma). They share a the western Mirdita massifs, Puka, and Krabbi (Fig. 1A insert) (Nicolas et al., CITATION: Nicolas, A., Meshi, A., Boudier, F., Jous- selin, D., and Muceku, B., 2017, Mylonites in ophiolite common horizontal Jurassic–Lower Cretaceous sedimentary cover showing 1999; Meshi et al., 2009). Other OCC candidates include Chenaillet (Manatschal of Mirdita (Albania): Oceanic detachment shear zone: that they were not deeply and intrinsically affected by later Alpine thrusting. et al., 2011) and Thetford Mine in eastern Canada, which has been compared to Geosphere, v. 13, no. 1, p. 136–154, doi: 10 .1130 The western massifs of Mirdita, first oceanic core complex (OCC) and detach- Mirdita (Tremblay et al., 2009).