Identification and Structure Activity Relationship of Small Molecule Antagonists
Total Page:16
File Type:pdf, Size:1020Kb
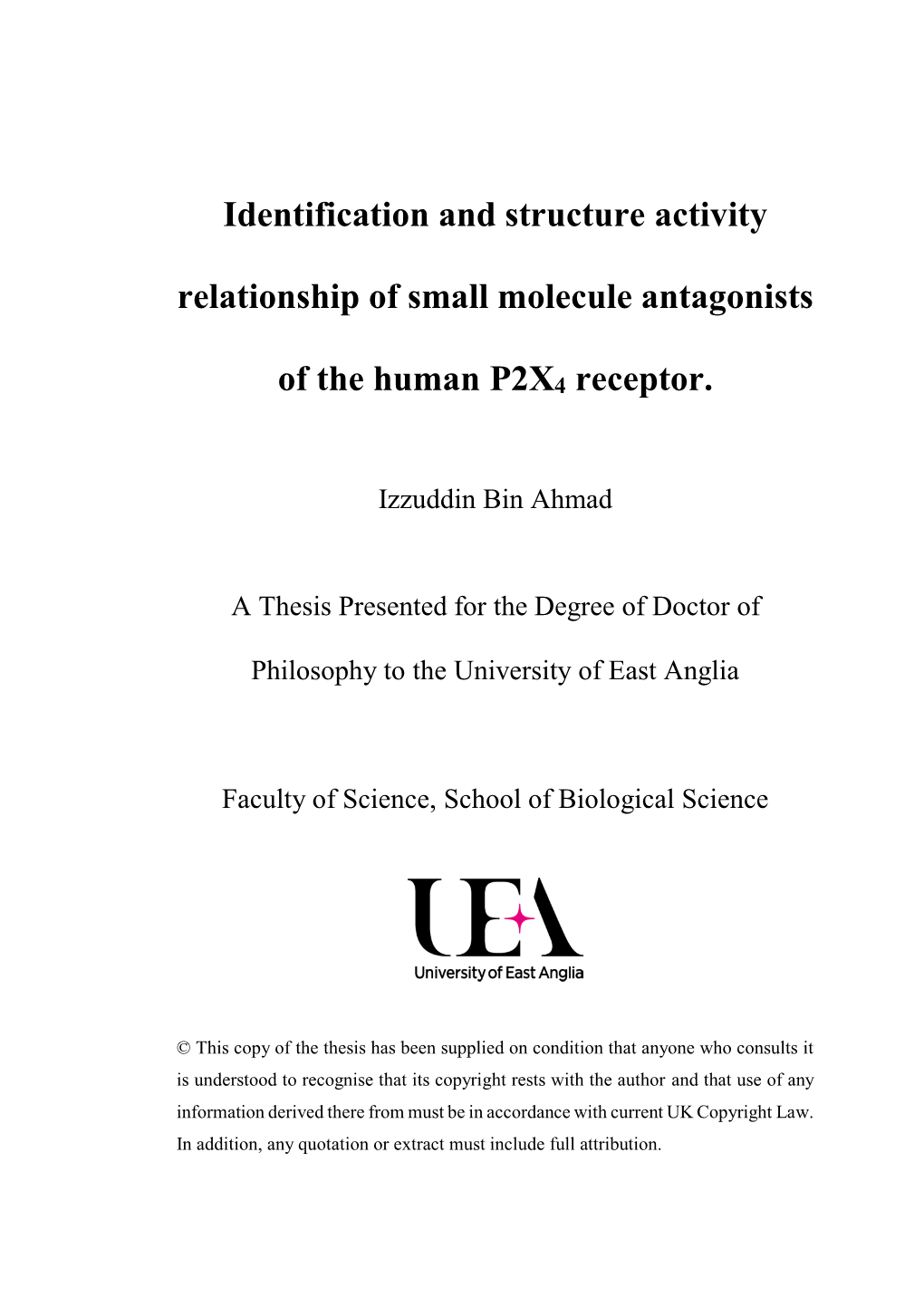
Load more
Recommended publications
-
The Bhagirathi Cooperative Milk Producers' Union Limited
The Bhagirathi Cooperative Milk Producers’ Union Limited TESTING PARAMETERS OF MILK & MILK PRODUCTS THAT SHOULD BE TESTED MICROBIOLOGICAL PARAMETER FOR PANEER 1] SPC(cfu/ ml) 2] Coliform (cfu/ ml) 3] E.Coli (cfu/ ml) 4] Salmonella (cfu/ 25gm) 5] Listeria monocytogenes (cfu/ gm) 6] Staphylococcus aureus (cfu/ gm) 7] Yeast & mould count (cfu/ gm) MICROBIOLOGICAL PARAMETER FOR DAHI 1] Coliform (cfu/ ml) 2] E.Coli (cfu/ ml) 3] Salmonella (cfu/ 25gm) 4] Listeria monocytogenes (cfu/ gm) 5] Staphylococcus aureus (cfu/ gm) 6] Yeast & mould count (cfu/ gm) 7] Anaerobic Spore count (cfu/ gm) MICROBIOLOGICAL PARAMETER IN FINISHED MILK THAT SHOULD BE TESTED 1] SPC(cfu/ ml) 2] Coliform (cfu/ ml) 3] Salmonella (cfu/ 25gm) 4] Listeria monocytogenes (cfu/ gm) The Bhagirathi Cooperative Milk Producers’ Union Limited LIST OF ANTIBIOTICS IN FINISHED MILK THAT SHOULD BE TESTED 1. Ampicillin 2. Cloxacillin 3. Colistin 4. Dihydrostreptomycin Streptomycin 5. Chlortetracycline/Oxytetracycline/Tetracycline 6. Lincomycin 7. Neomycin 8. Salinomycin 9. Spectinomycin 10. Sulphadiazine 11. Sulphathiazole Sodium 12. Trimethoprim 13. Sulfadiazine 14. Sulfanilamide 15. Sulfaguanidine 16. Zine Bacitracin (minimum 60lU/mg dried substance) 17. Amprolium 18. Apramycin 19. Ceftiofur 20. Cephapirine 21. Clopidol 22. Enrofloxacin 23. Ethopabate 24. Flavophospholipol (Flavomycin) 25. Monensin 26. Sulphaquinoxaline 27. Sulfadimidine 28. Tyvalosin Tartrate 29. Virginiamycin 30. Acepromazine 31. Albendazole 32. Amitraz 33. Aspirin 34. Buserelin 35. Butafosfane 36. Butaphosphan 37. Calcium Borogluconate 38. Calcium Magnesium Borogluconate 39. Carboprost tromethamine 40. Cefquinone Sulphate 41. Chloral hydrate 42. Closprostenol Sodium 43. Clenbutrol (Broncopulmin powder) 44. Diethylcarbarnazine 45. Dinitolmide 46. Doramectin The Bhagirathi Cooperative Milk Producers’ Union Limited LIST OF ANTIBIOTICS IN FINISHED MILK THAT SHOULD BE TESTED 47. -
Ion Channels
UC Davis UC Davis Previously Published Works Title THE CONCISE GUIDE TO PHARMACOLOGY 2019/20: Ion channels. Permalink https://escholarship.org/uc/item/1442g5hg Journal British journal of pharmacology, 176 Suppl 1(S1) ISSN 0007-1188 Authors Alexander, Stephen PH Mathie, Alistair Peters, John A et al. Publication Date 2019-12-01 DOI 10.1111/bph.14749 License https://creativecommons.org/licenses/by/4.0/ 4.0 Peer reviewed eScholarship.org Powered by the California Digital Library University of California S.P.H. Alexander et al. The Concise Guide to PHARMACOLOGY 2019/20: Ion channels. British Journal of Pharmacology (2019) 176, S142–S228 THE CONCISE GUIDE TO PHARMACOLOGY 2019/20: Ion channels Stephen PH Alexander1 , Alistair Mathie2 ,JohnAPeters3 , Emma L Veale2 , Jörg Striessnig4 , Eamonn Kelly5, Jane F Armstrong6 , Elena Faccenda6 ,SimonDHarding6 ,AdamJPawson6 , Joanna L Sharman6 , Christopher Southan6 , Jamie A Davies6 and CGTP Collaborators 1School of Life Sciences, University of Nottingham Medical School, Nottingham, NG7 2UH, UK 2Medway School of Pharmacy, The Universities of Greenwich and Kent at Medway, Anson Building, Central Avenue, Chatham Maritime, Chatham, Kent, ME4 4TB, UK 3Neuroscience Division, Medical Education Institute, Ninewells Hospital and Medical School, University of Dundee, Dundee, DD1 9SY, UK 4Pharmacology and Toxicology, Institute of Pharmacy, University of Innsbruck, A-6020 Innsbruck, Austria 5School of Physiology, Pharmacology and Neuroscience, University of Bristol, Bristol, BS8 1TD, UK 6Centre for Discovery Brain Science, University of Edinburgh, Edinburgh, EH8 9XD, UK Abstract The Concise Guide to PHARMACOLOGY 2019/20 is the fourth in this series of biennial publications. The Concise Guide provides concise overviews of the key properties of nearly 1800 human drug targets with an emphasis on selective pharmacology (where available), plus links to the open access knowledgebase source of drug targets and their ligands (www.guidetopharmacology.org), which provides more detailed views of target and ligand properties. -
Poster Munoz Et Al Avm 4 Nt
ANTHELMINTIC AVERMECTINS FOR THE TREATMENT OF NON- TUBERCULOSIS MYCOBACTERIA INFECTIONS IN CYSTIC FIBROSIS Lara Muñoz Muñoz1,2,*, Charles J. Thompson3, and Santiago Ramón-García2,3,4,* 1 Clinical University Hospital Lozano Blesa, Zaragoza, Spain 2 Department of Microbiology, Preventive Medicine and Public Health, Faculty of Medicine, University of Zaragoza, Spain; 3 Department of Microbiology and Immunology, Centre for Tuberculosis Research, University of British Columbia, Canada; 4 Research & Development Agency of Aragon (ARAID) Foundation, Spain. *Email: [email protected] and [email protected] INTRODUCTION Pulmonary disease caused by non-tuberculosis mycobacteria (NTM) has emerged as a major threat to the health of individuals with cystic fibrosis (CF). The NTM most commonly identified are Mycobacterium abscessus (MABSC) and Mycobacterium avium (MAC) complexes. MABSC includes 3 species M. abscessus sb. abscessus, M. abscessus sb. bolletii and M. abscessus sb. masiliense. Ivermectin Selamectin Avermectins are a family of macrocyclic lactone compounds used as anthelmintics. Although inactive against Gram-positive and Gram-negative bacteria, they have demonstrated in vitro activity against mycobacterial species, including Mycobacterium tuberculosis, Mycobacterium ulcerans and Mycobacteriym marinum (PMID: 26270480 & 23165468). Milbemycin Doramectin OBJECTIVE oxime To evaluate the in vitro activity of the avermectins against MABSC and MAC. CONCLUSIONS The avermectins comprise clinically approved drugs (i.e. ivermectin) and are extensively -
Sheet1 Page 1 a Abamectin Acetazolamide Sodium Adenosine-5-Monophosphate Aklomide Albendazole Alfaxalone Aloe Vera Alphadolone A
Sheet1 A Abamectin Acetazolamide sodium Adenosine-5-monophosphate Aklomide Albendazole Alfaxalone Aloe vera Alphadolone Acetate Alpha-galactosidase Altrenogest Amikacin and its salts Aminopentamide Aminopyridine Amitraz Amoxicillin Amphomycin Amphotericin B Ampicillin Amprolium Anethole Apramycin Asiaticoside Atipamezole Avoparcin Azaperone B Bambermycin Bemegride Benazepril Benzathine cloxacillin Benzoyl Peroxide Benzydamine Bephenium Bephenium Hydroxynaphthoate Betamethasone Boldenone undecylenate Boswellin Bromelain Bromhexine 2-Bromo-2-nitropan-1, 3 diol Bunamidine Buquinolate Butamisole Butonate Butorphanol Page 1 Sheet1 C Calcium glucoheptonate (calcium glucoheptogluconate) Calcium levulinate Cambendazole Caprylic/Capric Acid Monoesters Carbadox Carbomycin Carfentanil Carnidazole Carnitine Carprofen Cefadroxil Ceftiofur sodium Centella asiatica Cephaloridine Cephapirin Chlorine dioxide Chlormadinone acetate Chlorophene Chlorothiazide Chlorpromazine HCl Choline Salicylate Chondroitin sulfate Clazuril Clenbuterol Clindamycin Clomipramine Clopidol Cloprostenol Clotrimazole Cloxacillin Colistin sulfate Copper calcium edetate Copper glycinate Coumaphos Cromolyn sodium Crystalline Hydroxycobalamin Cyclizine Cyclosporin A Cyprenorphine HCl Cythioate D Decoquinate Demeclocycline (Demethylchlortetracycline) Page 2 Sheet1 Deslorelin Desoxycorticosterone Pivalate Detomidine Diaveridine Dichlorvos Diclazuril Dicloxacillin Didecyl dimethyl ammonium chloride Diethanolamine Diethylcarbamazine Dihydrochlorothiazide Diidohydroxyquin Dimethylglycine -
Recommended Classification of Pesticides by Hazard and Guidelines to Classification 2019 Theinternational Programme on Chemical Safety (IPCS) Was Established in 1980
The WHO Recommended Classi cation of Pesticides by Hazard and Guidelines to Classi cation 2019 cation Hazard of Pesticides by and Guidelines to Classi The WHO Recommended Classi The WHO Recommended Classi cation of Pesticides by Hazard and Guidelines to Classi cation 2019 The WHO Recommended Classification of Pesticides by Hazard and Guidelines to Classification 2019 TheInternational Programme on Chemical Safety (IPCS) was established in 1980. The overall objectives of the IPCS are to establish the scientific basis for assessment of the risk to human health and the environment from exposure to chemicals, through international peer review processes, as a prerequisite for the promotion of chemical safety, and to provide technical assistance in strengthening national capacities for the sound management of chemicals. This publication was developed in the IOMC context. The contents do not necessarily reflect the views or stated policies of individual IOMC Participating Organizations. The Inter-Organization Programme for the Sound Management of Chemicals (IOMC) was established in 1995 following recommendations made by the 1992 UN Conference on Environment and Development to strengthen cooperation and increase international coordination in the field of chemical safety. The Participating Organizations are: FAO, ILO, UNDP, UNEP, UNIDO, UNITAR, WHO, World Bank and OECD. The purpose of the IOMC is to promote coordination of the policies and activities pursued by the Participating Organizations, jointly or separately, to achieve the sound management of chemicals in relation to human health and the environment. WHO recommended classification of pesticides by hazard and guidelines to classification, 2019 edition ISBN 978-92-4-000566-2 (electronic version) ISBN 978-92-4-000567-9 (print version) ISSN 1684-1042 © World Health Organization 2020 Some rights reserved. -
(12) United States Patent (10) Patent No.: US 9,173.403 B2 Rosentel, Jr
USOO9173403B2 (12) United States Patent (10) Patent No.: US 9,173.403 B2 Rosentel, Jr. et al. (45) Date of Patent: Nov. 3, 2015 (54) PARASITICIDAL COMPOSITIONS FOREIGN PATENT DOCUMENTS COMPRISING MULTIPLE ACTIVE AGENTS, BR PIO403620 A 3, 2006 METHODS AND USES THEREOF EP 83.6851 A 4f1998 GB 2457734 8, 2009 (75) Inventors: Joseph K. Rosentel, Jr., Johns Creek, WO WO 98,17277 4f1998 GA (US); Monica Tejwani, Monmouth WO WO O2/O94233 11, 2002 WO WO2004/O16252 2, 2004 Junction, NJ (US); Arima Das-Nandy, WO WO 2007/O18659 2, 2007 Titusville, NJ (US) WO WO 2008/O3O385 3, 2008 WO 2008/136791 11, 2008 (73) Assignee: MERLAL, INC., Duluth, GA (US) WO WO 2009/O18198 2, 2009 WO WO 2009/027506 3, 2009 WO 2009/112837 9, 2009 (*) Notice: Subject to any disclaimer, the term of this WO WO 2010/026370 3, 2010 patent is extended or adjusted under 35 WO WO2010.109214 9, 2010 U.S.C. 154(b) by 100 days. OTHER PUBLICATIONS (21) Appl. No.: 13/078,496 Notice of Opposition in the matter of New Zealand Patent Applica (22) Filed: Apr. 1, 2011 tion 595934 in the name of Norbrook Laboratories Limited and Opposition thereto by Merial Limited dated Jun. 28, 2014. (65) Prior Publication Data First Supplementary Notice of Opposition in the matter of New Zealand Patent Application 595934 in the name of Norbrook Labo US 2011 FO245191 A1 Oct. 6, 2011 ratories Limited and Opposition thereto by Merial Limited dated Aug. 28, 2014. Second Supplementary Notice of Opposition in the matter of New Related U.S. -
Recognition and Management of Pesticide Poisonings
HIGHLIGHTS CHAPTER 8 Derived from living systems Bacillus thuringiensis is the most important live agent Biologicals and Insecticides Generally of low-order of Biological Origin toxicity Poison control center advice can help avoid potentially harmful treatment This chapter concerns several widely used insecticidal products of natural origin, and also certain agents usually identified as biological control agents. This latter group includes many living control agents, though only the bacterial agent Bacillus thuringi- SIGNS & SYMPTOMS ensis will be discussed in detail, as it is one of the most widely used. Other agents, such as parasitic wasps and insects, are so host specific they pose little or no risk to man. Highly variable based on Many of the pesticides in this chapter, with the notable exception of nicotine, are specific agents relatively less toxic to mammals than to insects. Consequently, there may be no findings Several cause GI irritation of toxicity following ingestion of these compounds. While clinicians should always consider calling their regional poison control center (1-800-222-1222) for advice on Nicotine may have serious any poisoning, it may be of particular value in the case of some of these biological CNS effects pesticides, where no treatment is warranted and poison control center advice can help Nicotine and sabadilla may avoid potentially harmful treatments. have cardiovascular effects Agents are presented in alphabetical order. TREATMENT AVERMECTIN Specific to the agent Source and Products Skin, eye, GI Avermectin and related products are synthetically derived from the toxin of the soil decontamination may be bacterium Streptomyces avermitilis. They are used for control of mites, fire ants (ant indicated bait stations) and other insects. -
China Releases New Maximum Residue Limits for Pesticides In
GB 2763-2016 THIS REPORT CONTAINS ASSESSMENTS OF COMMODITY AND TRADE ISSUES MADE BY USDA STAFF AND NOT NECESSARILY STATEMENTS OF OFFICIAL U.S. GOVERNMENT POLICY Voluntary - Public Date: 3/31/2017 GAIN Report Number: CH17016 China - Peoples Republic of Post: Beijing China Releases New Maximum Residue Limits for Pesticides in Food Report Categories: FAIRS Subject Report Approved By: Lisa Anderson Prepared By: FAS Staff Report Highlights: On December 18, 2016, the Chinese National Health and Family Planning Commission, Ministry of Agriculture, China Food and Drug Administration released the National Food Safety Standard - Maximum Residue Limits for Pesticides in Foods (GB 2763-2016). The standard will replace the current MRL Standard (GB 2763-2014) and will be implemented on June 18, 2017. This report provides an unofficial translation of the standard. Editors’ Note: The asterisk appearing in the MRL column means that the limit is a temporary MRL. A temporary MRL is usually set under the following four conditions: 1. The dietary risk assessment data is incomplete; 2. The Acceptable Daily Intake (ADI) is temporary (ADI is used as the basis for MRL setting); 3. There is no surveillance or analysis method for the MRL that complies with the standard requirements; 4. In emergency situations, the pesticide is approved to be used on un-registered crops. I GB 2763-2016 General Information: BEGIN TRANSLATION ICS 65.100 G 25 GB National Standard of the People’s Republic of China GB 2763—2016 Replacing GB 2763 - 2014 National food safety standard Maximum Residue Limits for Pesticides in Food General Information: National Health and Family Planning Commission Issued by: Ministry of Agriculture China Food and Drug Administration Issued on: 2016-12-18 Implementation:2017-06-18 II GB 2763-2016 Table of Content Preface ............................................................................................................................................................... -
Tolerance and Efficacy of Emamectin Benzoate and Ivermectin for the Treatment of Pseudocapillaria Tomentosa in Laboratory Zebrafish (Danio Rerio)
Tolerance and Efficacy of Emamectin Benzoate and Ivermectin for the Treatment of Pseudocapillaria tomentosa in Laboratory Zebrafish (Danio rerio) Collymore, C., Watral, V., White, J. R., Colvin, M. E., Rasmussen, S., Tolwani, R. J., & Kent, M. L. (2014). Tolerance and Efficacy of Emamectin Benzoate and Ivermectin for the Treatment of Pseudocapillaria tomentosa in Laboratory Zebrafish (Danio rerio). Zebrafish, 11(5), 490-497. doi:10.1089/zeb.2014.1021 10.1089/zeb.2014.1021 Mary Ann Liebert, Inc. Version of Record http://cdss.library.oregonstate.edu/sa-termsofuse ZEBRAFISH Volume 11, Number 5, 2014 Fish Haus ª Mary Ann Liebert, Inc. DOI: 10.1089/zeb.2014.1021 Tolerance and Efficacy of Emamectin Benzoate and Ivermectin for the Treatment of Pseudocapillaria tomentosa in Laboratory Zebrafish (Danio rerio) Chereen Collymore,1,* Virginia Watral,2 Julie R. White,1,3 Michael E. Colvin,2 Skye Rasmussen,1,3 Ravi J. Tolwani,1,3 and Michael L. Kent2 Abstract Tolerance of adult zebrafish and efficacy of emamectin benzoate and ivermectin in eliminating Pseudoca- pillaria tomentosa infection were evaluated. In the tolerance study, behavioral changes, fecundity, histopa- thology, and mortality were evaluated for in-feed administration of emamectin (0.05, 0.10, and 0.25 mg/kg) and ivermectin (0.05 and 0.10 mg/kg). All doses of emamectin were well tolerated. Ivermectin 0.05 mg/kg ad- ministration resulted in mild behavioral changes and a transient decrease in fecundity. Ivermectin 0.10 mg/kg administration resulted in severe behavioral changes and some mortality. In the efficacy study, emamectin (0.05 and 0.25 mg/kg) and ivermectin (0.05 mg/kg) were evaluated for their efficacy in eliminating P. -
DETERMINATION of BENZIMIDAZOLE and AVERMECTIN RESIDUES in BOVINE MILK by LIQUID CHROMATOGRAPHY -TANDEM MASS SPECTROMETRY Date
DETERMINATION OF BENZIMIDAZOLE AND AVERMECTIN RESIDUES IN BOVINE MILK BY LIQUID CHROMATOGRAPHY -TANDEM MASS SPECTROMETRY Date: 2014-april-18 PRINCIPLE This method is based on the principle of the quick, easy, cheap, effective, rugged and safe (QuEChERS) method [1]. It includes extraction of a representative portion of the sample with acetonitrile (MeCN) followed by salting out and dispersive solid-phase extraction with a mixture of magnesium sulphate and C18 material. After clean-up, an aliquot of the supernatant is analyzed by liquid chromatography tandem mass spectrometry (LC-MS/MS). SCOPE This analytical method includes determination of residues of seven benzimidazoles (albendazole, thiabendazole, albendazole-sulphoxide, albendazole-sulphone, triclabendazole, triclabendazole-sulphoxide and triclabendazole-sulphone) and three avermectins (abamectin, emamectin and ivermectin) in bovine milk at concentration levels of 5 ngg−1 to 500 ngg−1. MATERIALS The following reagents and chemical are applicable: MeCN; High performance liquid chromatography (HPLC) grade Methanol (MeOH); Octadecylsilane sorbent C18; Sodium Chloride (NaCl), analytical grade; Ammonium acetate, analytical grade; Formic acid, analytical grade; Primary secondary amine (PSA) sorbent; Magnesium sulphate anhydrous. Standards and stock solutions The analytical standards include: Albendazole 99.6% and Ivermectin 91.0% from United States Pharmacopoeia (USP); Thiabendazole 98.3%, Abamectin 94.4%, Emamectin, 96.5%; Cyprodinil, 99.5% all from Chem service; Albendazole-sulphoxide, -
GM1 Signaling Through the GDNF Receptor
Zebrafish Neuronal Nicotinic Acetylcholine Receptors: Cloning, Expression, and Functional Analysis DISSERTATION Presented in Partial Fulfillment of the Requirements for the Degree Doctor of Philosophy in the Graduate School of The Ohio State University By Kristin Michelle Ackerman ***** The Ohio State University 2009 Dissertation Committee: Approved by Professor Robert Thomas Boyd, Advisor Professor Christine Beattie _________________________________ Professor Dennis McKay Advisor Professor James Jontes Integrated Biomedical Sciences ABSTRACT Cigarette smoking is a major public health concern, in that, over one billion people or one in three adults in the world smoke (WHO, 2006). Even more alarming is that approximately 20% of pregnant women in the western world continue to smoke during pregnancy despite a number of fetal complications linked with cigarette smoking (CDC, 2004, 2006 and U.S. Surgeon General’s Report (Chapter 5; pages 180–194). Smoking during pregnancy can cause serious health problems for both mother and child, such as pregnancy complications, premature birth, low-birth-weight infants, stillbirth and infant death (U.S. Department of Health and Human Services, 2004). Nicotine exposure in utero can also result in long-term anatomical, behavioral and cognitive deficits (Sexton et al., 1990; Olds et al., 1994; Slotkin 1992, 2004, 2008). Nicotine, the main addictive chemical component in cigarette tobacco, is a likely contributor to the adverse outcomes, as fetal brain and spinal cord are primary targets. Nicotine mediates its actions through nicotinic acetylcholine receptors, nAChRs, which are widely distributed throughout the body. This class of receptors belongs to the ligand gated ion channel superfamily, which includes GABA A, glycine, 5-HT3, and NMDA-type glutamate receptors. -
NIH Public Access Author Manuscript Neurotoxicology
NIH Public Access Author Manuscript Neurotoxicology. Author manuscript; available in PMC 2012 October 1. NIH-PA Author ManuscriptPublished NIH-PA Author Manuscript in final edited NIH-PA Author Manuscript form as: Neurotoxicology. 2011 October ; 32(5): 661±665. doi:10.1016/j.neuro.2011.06.002. Channelopathies: Summary of the Hot Topic Keynotes Session Jason P. Magby1, April P. Neal2, William D. Atchison2, Isaac P. Pessah3, and Timothy J. Shafer4 1Joint Graduate Program in Toxicology, Rutgers University / University of Medicine and Dentistry New Jersey 170 Frelinghuysen Rd. Piscataway, NJ 2Department of Pharmacology and Toxicology, Michigan State University, East Lansing, Michigan 3Department of Molecular Biosciences, School of Veterinary Medicine, University of California, Davis, CA 4Integrated Systems Toxicology Division, National Health and Environmental Effects Research Laboratory, Office of Research and Development, US Environmental Protection Agency, Research Triangle Park, NC, USA The “Hot Topic Keynotes: Channelopathies” session of the 26th International Neurotoxicology Conference brought together toxicologists studying interactions of environmental toxicants with ion channels, to review the state of the science of channelopathies and to discuss the potential for interactions between environmental exposures and channelopathies. This session presented an overview of chemicals altering ion channel function and background about different channelopathy models. It then explored the available evidence that individuals with channelopathies may or may not be more sensitive to effects of chemicals. Dr. Tim Shafer began his presentation by defining what channelopathies are and presenting several examples of channelopathies. Channelopathies are mutations that alter the function of ion channels such that they result in clinically-definable syndromes including forms of epilepsy, migraine headache, ataxia and other neurological and cardiac syndromes (Kullmann, 2010).