Geomorphological Effectiveness of Floods to Rework Gravel Bars: Insight from Hyperscale Topography and Hydraulic Modelling
Total Page:16
File Type:pdf, Size:1020Kb
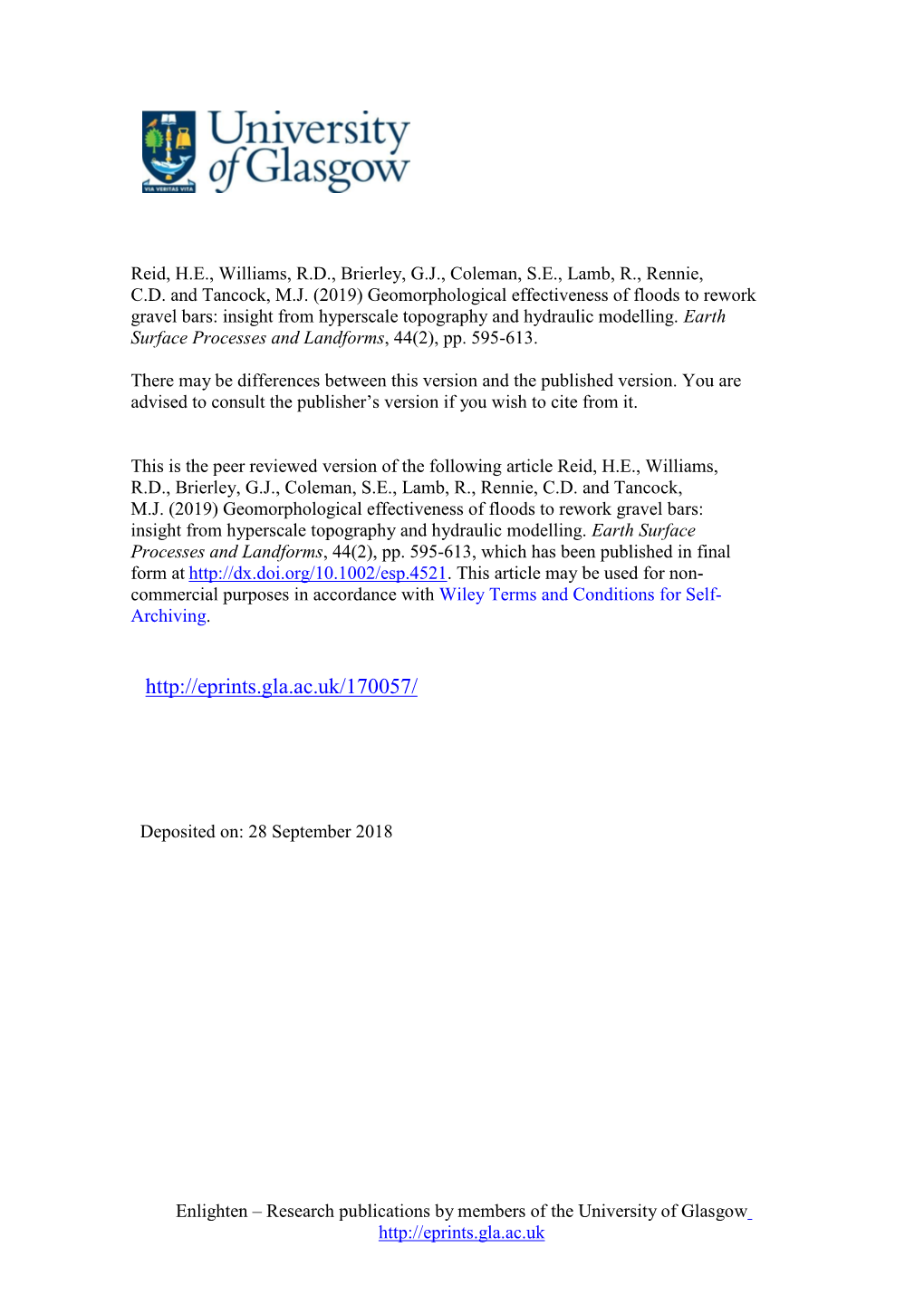
Load more
Recommended publications
-
(P 117-140) Flood Pulse.Qxp
117 THE FLOOD PULSE CONCEPT: NEW ASPECTS, APPROACHES AND APPLICATIONS - AN UPDATE Junk W.J. Wantzen K.M. Max-Planck-Institute for Limnology, Working Group Tropical Ecology, P.O. Box 165, 24302 Plön, Germany E-mail: [email protected] ABSTRACT The flood pulse concept (FPC), published in 1989, was based on the scientific experience of the authors and published data worldwide. Since then, knowledge on floodplains has increased considerably, creating a large database for testing the predictions of the concept. The FPC has proved to be an integrative approach for studying highly diverse and complex ecological processes in river-floodplain systems; however, the concept has been modified, extended and restricted by several authors. Major advances have been achieved through detailed studies on the effects of hydrology and hydrochemistry, climate, paleoclimate, biogeography, biodi- versity and landscape ecology and also through wetland restoration and sustainable management of flood- plains in different latitudes and continents. Discussions on floodplain ecology and management are greatly influenced by data obtained on flow pulses and connectivity, the Riverine Productivity Model and the Multiple Use Concept. This paper summarizes the predictions of the FPC, evaluates their value in the light of recent data and new concepts and discusses further developments in floodplain theory. 118 The flood pulse concept: New aspects, INTRODUCTION plain, where production and degradation of organic matter also takes place. Rivers and floodplain wetlands are among the most threatened ecosystems. For example, 77 percent These characteristics are reflected for lakes in of the water discharge of the 139 largest river systems the “Seentypenlehre” (Lake typology), elaborated by in North America and Europe is affected by fragmen- Thienemann and Naumann between 1915 and 1935 tation of the river channels by dams and river regula- (e.g. -
A 3D Forward Stratigraphic Model of Fluvial Meander-Bend Evolution for Prediction of Point-Bar Lithofacies Architecture
This is a repository copy of A 3D forward stratigraphic model of fluvial meander-bend evolution for prediction of point-bar lithofacies architecture. White Rose Research Online URL for this paper: http://eprints.whiterose.ac.uk/116031/ Version: Accepted Version Article: Yan, N orcid.org/0000-0003-1790-5861, Mountney, NP orcid.org/0000-0002-8356-9889, Colombera, L et al. (1 more author) (2017) A 3D forward stratigraphic model of fluvial meander-bend evolution for prediction of point-bar lithofacies architecture. Computers & Geosciences, 105. pp. 65-80. ISSN 0098-3004 https://doi.org/10.1016/j.cageo.2017.04.012 © 2017 Elsevier Ltd. This manuscript version is made available under the CC-BY-NC-ND 4.0 license http://creativecommons.org/licenses/by-nc-nd/4.0/ Reuse Items deposited in White Rose Research Online are protected by copyright, with all rights reserved unless indicated otherwise. They may be downloaded and/or printed for private study, or other acts as permitted by national copyright laws. The publisher or other rights holders may allow further reproduction and re-use of the full text version. This is indicated by the licence information on the White Rose Research Online record for the item. Takedown If you consider content in White Rose Research Online to be in breach of UK law, please notify us by emailing [email protected] including the URL of the record and the reason for the withdrawal request. [email protected] https://eprints.whiterose.ac.uk/ Author’s Accepted Manuscript A 3D forward stratigraphic model of fluvial meander-bend evolution for prediction of point-bar lithofacies architecture Na Yan, Nigel P. -
Calvin Park Tributary Stream Restoration -- Survey Phase
Neighborhood Advisory Croydon Creek – Calvin Park Tributary Stream Restoration -- Survey Phase Project Rockville’s Department of Public Works has begun the design of the Croydon Creek – Description: Calvin Park Tributary Stream Restoration project. The city’s consultant, AECOM, will survey the area within Rockville Civic Center Park in the project limits depicted on the back side of this advisory. The survey will identify features including the existing roads, stream, trees, utilities, and topography. Survey work will begin in March and will continue into the spring. Surveyors and engineers with equipment will traverse the area shown on the back of this advisory. Survey stakes may be placed in the ground and trees may be marked with temporary metal tags to assist in identification and inventory. The survey flags do not indicate tree removal. Purpose: The Croydon Creek – Calvin Park Tributary Stream Restoration project was recommended in the 2013 Rock Creek Watershed Assessment as a crucial component to the long-term health of the watershed. The assessment was approved by the Mayor and Council in February 2013, and this project was included in the adopted Fiscal Year 2017 Capital Improvements Program. The project goals are to enhance the watershed through stream restoration, wetland enhancement, reforestation, and the protection of private and public property and infrastructure Location: Croydon Creek within Rockville Civic Center Park and the Calvin Park Tributary from Baltimore Road to Croydon Creek (See the map on the back of this advisory). Timeline: Survey work and preliminary design is anticipated to begin in March and continue through spring. A community meeting will be held this summer to provide additional details of the project. -
Measurement of Bedload Transport in Sand-Bed Rivers: a Look at Two Indirect Sampling Methods
Published online in 2010 as part of U.S. Geological Survey Scientific Investigations Report 2010-5091. Measurement of Bedload Transport in Sand-Bed Rivers: A Look at Two Indirect Sampling Methods Robert R. Holmes, Jr. U.S. Geological Survey, Rolla, Missouri, United States. Abstract Sand-bed rivers present unique challenges to accurate measurement of the bedload transport rate using the traditional direct sampling methods of direct traps (for example the Helley-Smith bedload sampler). The two major issues are: 1) over sampling of sand transport caused by “mining” of sand due to the flow disturbance induced by the presence of the sampler and 2) clogging of the mesh bag with sand particles reducing the hydraulic efficiency of the sampler. Indirect measurement methods hold promise in that unlike direct methods, no transport-altering flow disturbance near the bed occurs. The bedform velocimetry method utilizes a measure of the bedform geometry and the speed of bedform translation to estimate the bedload transport through mass balance. The bedform velocimetry method is readily applied for the estimation of bedload transport in large sand-bed rivers so long as prominent bedforms are present and the streamflow discharge is steady for long enough to provide sufficient bedform translation between the successive bathymetric data sets. Bedform velocimetry in small sand- bed rivers is often problematic due to rapid variation within the hydrograph. The bottom-track bias feature of the acoustic Doppler current profiler (ADCP) has been utilized to accurately estimate the virtual velocities of sand-bed rivers. Coupling measurement of the virtual velocity with an accurate determination of the active depth of the streambed sediment movement is another method to measure bedload transport, which will be termed the “virtual velocity” method. -
Deposition Patterns and Rates of Mining-Contaminated Sediment Within a Sedimentation Basin System, S.E
BearWorks Institutional Repository MSU Graduate Theses Spring 2017 Deposition Patterns and Rates of Mining- Contaminated Sediment within a Sedimentation Basin System, S.E. Missouri Joshua Carl Voss Missouri State University - Springfield, [email protected] Follow this and additional works at: http://bearworks.missouristate.edu/theses Part of the Environmental Indicators and Impact Assessment Commons, Environmental Monitoring Commons, and the Hydrology Commons Recommended Citation Voss, Joshua Carl, "Deposition Patterns and Rates of Mining-Contaminated Sediment within a Sedimentation Basin System, S.E. Missouri" (2017). MSU Graduate Theses. 3074. http://bearworks.missouristate.edu/theses/3074 This article or document was made available through BearWorks, the institutional repository of Missouri State University. The orkw contained in it may be protected by copyright and require permission of the copyright holder for reuse or redistribution. For more information, please contact [email protected]. DEPOSITION PATTERNS AND RATES OF MINING-CONTAMINATED SEDIMENT WITHIN A SEDIMENTATION BASIN SYSTEM, BIG RIVER, S.E. MISSOURI A Masters Thesis Presented to The Graduate College of Missouri State University ATE In Partial Fulfillment Of the Requirements for the Degree Master of Science, Geospatial Sciences in Geography, Geology, and Planning By Josh C. Voss May 2017 Copyright 2017 by Joshua Carl Voss ii DEPOSITION PATTERNS AND RATES OF MINING-CONTAMINATED SEDIMENT WITHIN A SEDIMENTATION BASIN SYSTEM, BIG RIVER, S.E. MISSOURI Geography, Geology, and Planning Missouri State University, May 2017 Master of Science Josh C. Voss ABSTRACT Flooding events exert a dominant control over the deposition and formation of floodplains. The rate at which floodplains form depends on flood magnitude, frequency, and duration, and associated sediment transport capacity and supply. -
Lesson 4: Sediment Deposition and River Structures
LESSON 4: SEDIMENT DEPOSITION AND RIVER STRUCTURES ESSENTIAL QUESTION: What combination of factors both natural and manmade is necessary for healthy river restoration and how does this enhance the sustainability of natural and human communities? GUIDING QUESTION: As rivers age and slow they deposit sediment and form sediment structures, how are sediments and sediment structures important to the river ecosystem? OVERVIEW: The focus of this lesson is the deposition and erosional effects of slow-moving water in low gradient areas. These “mature rivers” with decreasing gradient result in the settling and deposition of sediments and the formation sediment structures. The river’s fast-flowing zone, the thalweg, causes erosion of the river banks forming cliffs called cut-banks. On slower inside turns, sediment is deposited as point-bars. Where the gradient is particularly level, the river will branch into many separate channels that weave in and out, leaving gravel bar islands. Where two meanders meet, the river will straighten, leaving oxbow lakes in the former meander bends. TIME: One class period MATERIALS: . Lesson 4- Sediment Deposition and River Structures.pptx . Lesson 4a- Sediment Deposition and River Structures.pdf . StreamTable.pptx . StreamTable.pdf . Mass Wasting and Flash Floods.pptx . Mass Wasting and Flash Floods.pdf . Stream Table . Sand . Reflection Journal Pages (printable handout) . Vocabulary Notes (printable handout) PROCEDURE: 1. Review Essential Question and introduce Guiding Question. 2. Hand out first Reflection Journal page and have students take a minute to consider and respond to the questions then discuss responses and questions generated. 3. Handout and go over the Vocabulary Notes. Students will define the vocabulary words as they watch the PowerPoint Lesson. -
Geomorphic Classification of Rivers
9.36 Geomorphic Classification of Rivers JM Buffington, U.S. Forest Service, Boise, ID, USA DR Montgomery, University of Washington, Seattle, WA, USA Published by Elsevier Inc. 9.36.1 Introduction 730 9.36.2 Purpose of Classification 730 9.36.3 Types of Channel Classification 731 9.36.3.1 Stream Order 731 9.36.3.2 Process Domains 732 9.36.3.3 Channel Pattern 732 9.36.3.4 Channel–Floodplain Interactions 735 9.36.3.5 Bed Material and Mobility 737 9.36.3.6 Channel Units 739 9.36.3.7 Hierarchical Classifications 739 9.36.3.8 Statistical Classifications 745 9.36.4 Use and Compatibility of Channel Classifications 745 9.36.5 The Rise and Fall of Classifications: Why Are Some Channel Classifications More Used Than Others? 747 9.36.6 Future Needs and Directions 753 9.36.6.1 Standardization and Sample Size 753 9.36.6.2 Remote Sensing 754 9.36.7 Conclusion 755 Acknowledgements 756 References 756 Appendix 762 9.36.1 Introduction 9.36.2 Purpose of Classification Over the last several decades, environmental legislation and a A basic tenet in geomorphology is that ‘form implies process.’As growing awareness of historical human disturbance to rivers such, numerous geomorphic classifications have been de- worldwide (Schumm, 1977; Collins et al., 2003; Surian and veloped for landscapes (Davis, 1899), hillslopes (Varnes, 1958), Rinaldi, 2003; Nilsson et al., 2005; Chin, 2006; Walter and and rivers (Section 9.36.3). The form–process paradigm is a Merritts, 2008) have fostered unprecedented collaboration potentially powerful tool for conducting quantitative geo- among scientists, land managers, and stakeholders to better morphic investigations. -
Seasonal Flooding Affects Habitat and Landscape Dynamics of a Gravel
Seasonal flooding affects habitat and landscape dynamics of a gravel-bed river floodplain Katelyn P. Driscoll1,2,5 and F. Richard Hauer1,3,4,6 1Systems Ecology Graduate Program, University of Montana, Missoula, Montana 59812 USA 2Rocky Mountain Research Station, Albuquerque, New Mexico 87102 USA 3Flathead Lake Biological Station, University of Montana, Polson, Montana 59806 USA 4Montana Institute on Ecosystems, University of Montana, Missoula, Montana 59812 USA Abstract: Floodplains are comprised of aquatic and terrestrial habitats that are reshaped frequently by hydrologic processes that operate at multiple spatial and temporal scales. It is well established that hydrologic and geomorphic dynamics are the primary drivers of habitat change in river floodplains over extended time periods. However, the effect of fluctuating discharge on floodplain habitat structure during seasonal flooding is less well understood. We collected ultra-high resolution digital multispectral imagery of a gravel-bed river floodplain in western Montana on 6 dates during a typical seasonal flood pulse and used it to quantify changes in habitat abundance and diversity as- sociated with annual flooding. We observed significant changes in areal abundance of many habitat types, such as riffles, runs, shallow shorelines, and overbank flow. However, the relative abundance of some habitats, such as back- waters, springbrooks, pools, and ponds, changed very little. We also examined habitat transition patterns through- out the flood pulse. Few habitat transitions occurred in the main channel, which was dominated by riffle and run habitat. In contrast, in the near-channel, scoured habitats of the floodplain were dominated by cobble bars at low flows but transitioned to isolated flood channels at moderate discharge. -
Sandbridge Beach FONSI
FINDING OF NO SIGNIFICANT IMPACT Issuance of a Negotiated Agreement for Use of Outer Continental Shelf Sand from Sandbridge Shoal in the Sandbridge Beach Erosion Control and Hurricane Protection Project Virginia Beach, Virginia Pursuant to the National Environmental Policy Act (NEPA), Council on Environmental Quality regulations implementing NEPA (40 CFR 1500-1508) and Department of the Interior (DOI) regulations implementing NEPA (43 CFR 46), the Bureau of Ocean Energy Management (BOEM) prepared an environmental assessment (EA) to determine whether the issuance of a negotiated agreement for the use of Outer Continental Shelf (OCS) sand from Sandbridge Shoal Borrow Areas A and B for the Sandbridge Beach Erosion Control and Hurricane Protection Project near Virginia Beach, VA would have a significant effect on the human environment and whether an environmental impact statement (EIS) should be prepared. Several NEPA documents evaluating impacts of the project have been previously prepared by both the US Army Corps of Engineers (USACE) and BOEM. The USACE described the affected environment, evaluated potential environmental impacts (initial construction and nourishment events), and considered alternatives to the proposed action in a 2009 EA. This EA was subsequently updated and adopted by BOEM in 2012 in association with the most recent 2013 Sandbridge nourishment effort (BOEM 2012). Prior to this, BOEM (previously Minerals Management Service [MMS]) was a cooperating agency on several EAs for previous projects (MMS 1997; MMS 2001; MMS 2006). This current EA, prepared by BOEM, supplements and summarizes the aforementioned 2012 analysis. BOEM has reviewed all prior analyses, supplemented additional information as needed, and determined that the potential impacts of the current proposed action have been adequately addressed. -
Sediment Transport and the Genesis Flood — Case Studies Including the Hawkesbury Sandstone, Sydney
Sediment Transport and the Genesis Flood — Case Studies including the Hawkesbury Sandstone, Sydney DAVID ALLEN ABSTRACT The rates at which parts of the geological record have formed can be roughly determined using physical sedimentology independently from other dating methods if current understanding of the processes involved in sedimentology are accurate. Bedform and particle size observations are used here, along with sediment transport equations, to determine rates of transport and deposition in various geological sections. Calculations based on properties of some very extensive rock units suggest that those units have been deposited at rates faster than any observed today and orders of magnitude faster than suggested by radioisotopic dating. Settling velocity equations wrongly suggest that rapid fine particle deposition is impossible, since many experiments and observations (for example, Mt St Helens, mud- flows) demonstrate that the conditions which cause faster rates of deposition than those calculated here are not fully understood. For coarser particles the only parameters that, when varied through reasonable ranges, very significantly affect transport rates are flow velocity and grain diameter. Popular geological models that attempt to harmonize the Genesis Flood with stratigraphy require that, during the Flood, most deposition believed to have occurred during the Palaeozoic and Mesozoic eras would have actually been the result of about one year of geological activity. Flow regimes required for the Flood to have deposited various geological cross- sections have been proposed, but the most reliable estimate of water velocity required by the Flood was attained for a section through the Tasman Fold Belt of Eastern Australia and equalled very approximately 30 ms-1 (100 km h-1). -
Sedimentation and Shoaling Work Unit
1 SEDIMENTARY PROCESSES lAND ENVIRONMENTS IIN THE COLUMBIA RIVER ESTUARY l_~~~~~~~~~~~~~~~7 I .a-.. .(.;,, . I _e .- :.;. .. =*I Final Report on the Sedimentation and Shoaling Work Unit of the Columbia River Estuary Data Development Program SEDIMENTARY PROCESSES AND ENVIRONMENTS IN THE COLUMBIA RIVER ESTUARY Contractor: School of Oceanography University of Washington Seattle, Washington 98195 Principal Investigator: Dr. Joe S. Creager School of Oceanography, WB-10 University of Washington Seattle, Washington 98195 (206) 543-5099 June 1984 I I I I Authors Christopher R. Sherwood I Joe S. Creager Edward H. Roy I Guy Gelfenbaum I Thomas Dempsey I I I I I I I - I I I I I I~~~~~~~~~~~~~~~~~~~~~~~~~~~~~~~~~~~~~~~~ PREFACE The Columbia River Estuary Data Development Program This document is one of a set of publications and other materials produced by the Columbia River Estuary Data Development Program (CREDDP). CREDDP has two purposes: to increase understanding of the ecology of the Columbia River Estuary and to provide information useful in making land and water use decisions. The program was initiated by local governments and citizens who saw a need for a better information base for use in managing natural resources and in planning for development. In response to these concerns, the Governors of the states of Oregon and Washington requested in 1974 that the Pacific Northwest River Basins Commission (PNRBC) undertake an interdisciplinary ecological study of the estuary. At approximately the same time, local governments and port districts formed the Columbia River Estuary Study Taskforce (CREST) to develop a regional management plan for the estuary. PNRBC produced a Plan of Study for a six-year, $6.2 million program which was authorized by the U.S. -
Topographic Forcing of Tidal Sandbar Patterns for Irregular Estuary Planforms
EARTH SURFACE PROCESSES AND LANDFORMS Earth Surf. Process. Landforms 43, 172–186 (2018) Copyright © 2017 John Wiley & Sons, Ltd. Published online 14 July 2017 in Wiley Online Library (wileyonlinelibrary.com) DOI: 10.1002/esp.4166 Topographic forcing of tidal sandbar patterns for irregular estuary planforms J. R. F. W. Leuven,* T. de Haas, L. Braat and M. G. Kleinhans Faculty of Geosciences, Utrecht University, Utrecht, The Netherlands Received 17 October 2016; Revised 17 April 2017; Accepted 19 April 2017 *Correspondence to: J. R. F. W. Leuven, Faculty of Geosciences, Utrecht University, Utrecht, The Netherlands. E-mail: [email protected] This is an open access article under the terms of the Creative Commons Attribution License, which permits use, distribution and reproduction in any medium, provided the original work is properly cited. ABSTRACT: Estuaries typically show converging planforms from the sea into the land. Nevertheless, their planform is rarely perfectly exponential and often shows curvature and the presence of embayments. Here we test the degree to which the shapes and dimensions of tidal sandbars depend on estuary planform. We assembled a dataset with 35 estuary planforms and properties of 190 tidal bars to induce broad-brush but significant empirical relations between channel planform, hydraulic geometry and bar pattern, and tested a linear stability theory for bar pattern. We found that the location where bars form is largely controlled by the excess width of a channel, which is calculated as the observed channel width minus the width of an ideal exponentially widening estuary. In general, the summed width of bars approximates the excess width as measured in the along-channel variation of three estuaries for which bathymetry was available as well as for the local measurements in the 35 investigated estuaries.