Heterotetrameric Channels of Kv2 and 'Silent' Kv Subunits
Total Page:16
File Type:pdf, Size:1020Kb
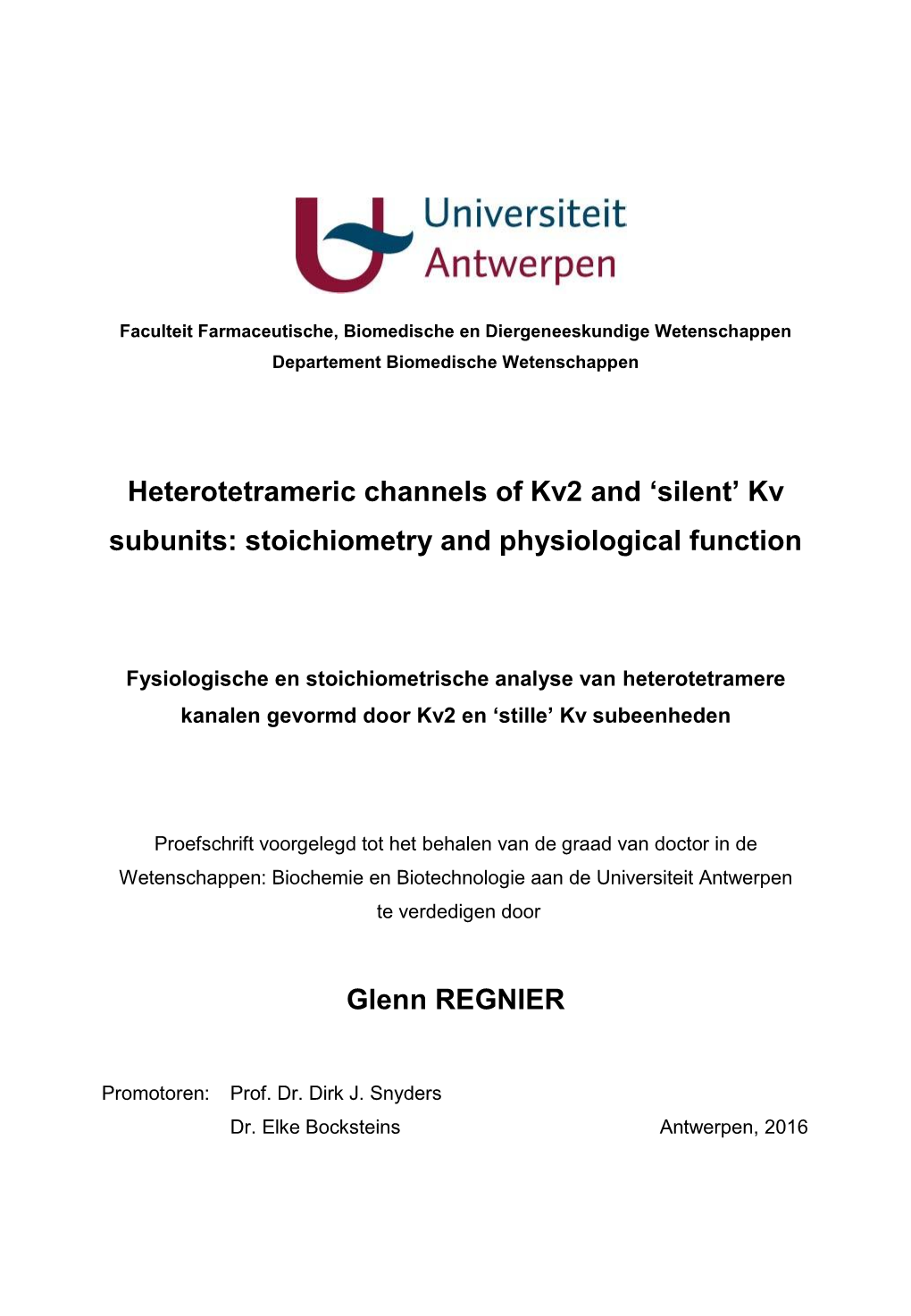
Load more
Recommended publications
-
Kv2 Dysfunction After Peripheral Axotomy Enhances Sensory Neuron
Our reference: YEXNR 11584 P-authorquery-v11 AUTHOR QUERY FORM Journal: YEXNR Please e-mail or fax your responses and any corrections to: Bennett, Timothy E-mail: [email protected] Fax: +1 619 699 6721 Article Number: 11584 Dear Author, Please check your proof carefully and mark all corrections at the appropriate place in the proof (e.g., by using on-screen annotation in the PDF file) or compile them in a separate list. Note: if you opt to annotate the file with software other than Adobe Reader then please also highlight the appropriate place in the PDF file. To ensure fast publication of your paper please return your corrections within 48 hours. For correction or revision of any artwork, please consult http://www.elsevier.com/artworkinstructions. Any queries or remarks that have arisen during the processing of your manuscript are listed below and highlighted by flags in the proof. Click on the ‘Q’ link to go to the location in the proof. Location in article Query / Remark: click on the Q link to go Please insert your reply or correction at the corresponding line in the proof Q1 Please confirm that given names and surnames have been identified correctly. Q2 Please check the layout of Table 5, and correct if necessary. Q3 A dummy footnote citation of footnote [**] has been inserted as a corresponding footnote text has been provided. Please check if the placement is correct and amend if necessary. Q4, Q5 This sentence has been slightly modified for clarity. Please check that the meaning is still correct, and amend if necessary. -
2012: Providence, Rhode Island
The 63rd Annual Meeting of the American Research Center in Egypt April 27-29, 2012 Renaissance Providence Hotel Providence, RI Photo Credits Front cover: Egyptian, Late Period, Saite, Dynasty 26 (ca. 664-525 BCE) Ritual rattle Glassy faience; h. 7 1/8 in Helen M. Danforth Acquisition Fund 1995.050 Museum of Art Rhode Island School of Design, Providence Photography by Erik Gould, courtesy of the Museum of Art, Rhode Island School of Design, Providence. Photo spread pages 6-7: Conservation of Euergates Gate Photo: Owen Murray Photo page 13: The late Luigi De Cesaris conserving paintings at the Red Monastery in 2011. Luigi dedicated himself with enormous energy to the suc- cess of ARCE’s work in cultural heritage preservation. He died in Sohag on December 19, 2011. With his death, Egypt has lost a highly skilled conservator and ARCE a committed colleague as well as a devoted friend. Photo: Elizabeth Bolman Abstracts title page 14: Detail of relief on Euergates Gate at Karnak Photo: Owen Murray Some of the images used in this year’s Annual Meeting Program Booklet are taken from ARCE conservation projects in Egypt which are funded by grants from the United States Agency for International Development (USAID). The Chronique d’Égypte has been published annually every year since 1925 by the Association Égyptologique Reine Élisabeth. It was originally a newsletter but rapidly became an international scientific journal. In addition to articles on various aspects of Egyptology, papyrology and coptology (philology, history, archaeology and history of art), it also contains critical reviews of recently published books. -
The Work of the Theban Mapping Project by Kent Weeks Saturday, January 30, 2021
Virtual Lecture Transcript: Does the Past Have a Future? The Work of the Theban Mapping Project By Kent Weeks Saturday, January 30, 2021 David A. Anderson: Well, hello, everyone, and welcome to the third of our January public lecture series. I'm Dr. David Anderson, the vice president of the board of governors of ARCE, and I want to welcome you to a very special lecture today with Dr. Kent Weeks titled, Does the Past Have a Future: The Work of the Theban Mapping Project. This lecture is celebrating the work of the Theban Mapping Project as well as the launch of the new Theban Mapping Project website, www.thebanmappingproject.com. Before we introduce Dr. Weeks, for those of you who are new to ARCE, we are a private nonprofit organization whose mission is to support the research on all aspects of Egyptian history and culture, foster a broader knowledge about Egypt among the general public and to support American- Egyptian cultural ties. As a nonprofit, we rely on ARCE members to support our work, so I want to first give a special welcome to our ARCE members who are joining us today. If you are not already a member and are interested in becoming one, I invite you to visit our website arce.org and join online to learn more about the organization and the important work that all of our members are doing. We provide a suite of benefits to our members including private members-only lecture series. Our next members-only lecture is on February 6th at 1 p.m. -
Protective Zika Vaccines Engineered to Eliminate Enhancement of Dengue Infection Via Immunodominance Switch
ARTICLES https://doi.org/10.1038/s41590-021-00966-6 Protective Zika vaccines engineered to eliminate enhancement of dengue infection via immunodominance switch Lianpan Dai 1,2,3,8 ✉ , Kun Xu3,8, Jinhe Li2,4,8, Qingrui Huang5, Jian Song 1, Yuxuan Han2, Tianyi Zheng2,4, Ping Gao2,4, Xuancheng Lu6, Huabing Yang 5, Kefang Liu7, Qianfeng Xia3, Qihui Wang 1, Yan Chai1, Jianxun Qi 1, Jinghua Yan 5 ✉ and George F. Gao 1,2,4 ✉ Antibody-dependent enhancement (ADE) is an important safety concern for vaccine development against dengue virus (DENV) and its antigenically related Zika virus (ZIKV) because vaccine may prime deleterious antibodies to enhance natural infections. Cross-reactive antibodies targeting the conserved fusion loop epitope (FLE) are known as the main sources of ADE. We design ZIKV immunogens engineered to change the FLE conformation but preserve neutralizing epitopes. Single vaccination conferred sterilizing immunity against ZIKV without ADE of DENV-serotype 1–4 infections and abrogated maternal–neonatal transmis- sion in mice. Unlike the wild-type-based vaccine inducing predominately cross-reactive ADE-prone antibodies, B cell profiling revealed that the engineered vaccines switched immunodominance to dispersed patterns without DENV enhancement. The crystal structure of the engineered immunogen showed the dimeric conformation of the envelope protein with FLE disruption. We provide vaccine candidates that will prevent both ZIKV infection and infection-/vaccination-induced DENV ADE. IKV is a mosquito-borne pathogen belonging to the genus models12,13,19,20. More importantly, a recent study of pediatric cohorts Flavivirus in the family Flaviviridae1. The 2015–2016 ZIKV in Nicaragua clearly shows that previous ZIKV infection enhanced epidemic spread to 84 countries worldwide, including future risk of DENV2 disease and severity, as well as DENV3 sever- Z2,3 21,22 China . -
Subunits Identified in the Human Genome
Obligatory heterotetramerization of three previously uncharacterized Kv channel ␣-subunits identified in the human genome N. Ottschytsch, A. Raes, D. Van Hoorick, and D. J. Snyders* Laboratory for Molecular Biophysics, Physiology, and Pharmacology, University of Antwerp (UIA) and Flanders Institute for Biotechnology (VIB), B2610 Antwerp, Belgium Edited by Lily Y. Jan, University of California School of Medicine, San Francisco, CA, and approved April 12, 2002 (received for review November 20, 2001) Voltage-gated K؉ channels control excitability in neuronal and sense, these ‘‘silent’’ subunits can be considered regulatory various other tissues. We identified three unique ␣-subunits of subunits—e.g., the metabolic regulation of the Kv2.1͞Kv9.3 -voltage-gated K؉-channels in the human genome. Analysis of the heteromultimer might play an important role in hypoxic pulmo full-length sequences indicated that one represents a previously nary artery vasoconstriction and in the possible development of uncharacterized member of the Kv6 subfamily, Kv6.3, whereas the pulmonary hypertension (8). others are the first members of two unique subfamilies, Kv10.1 and In this study we report the cloning and functional properties Kv11.1. Although they have all of the hallmarks of voltage-gated of three previously uncharacterized subunits that were identified K؉ channel subunits, they did not produce K؉ currents when in the early public draft version of the human genome. Based on expressed in mammalian cells. Confocal microscopy showed that sequence identity, one of these is a previously uncharacterized Kv6.3, Kv10.1, and Kv11.1 alone did not reach the plasma mem- member of the Kv6 subfamily (Kv6.3), whereas the others are the brane, but were retained in the endoplasmic reticulum. -
I General for Place Names See Also Maps and Their Keys
Cambridge University Press 978-0-521-12098-2 - Ancient Egyptian Materials and Technology Edited by Paul T. Nicholson and Ian Shaw Index More information Index I General For place names see also maps and their keys. AAS see atomic absorption specrophotometry Tomb E21 52 aerenchyma 229 Abbad region 161 Tomb W2 315 Aeschynomene elaphroxylon 336 Abdel ‘AI, 1. 51 Tomb 113 A’09 332 Afghanistan 39, 435, 436, 443 abesh 591 Umm el-Qa’ab, 63, 79, 363, 496, 577, 582, African black wood 338–9, 339 Abies 445 591, 594, 631, 637 African iron wood 338–9, 339 A. cilicica 348, 431–2, 443, 447 Tomb Q 62 agate 15, 21, 25, 26, 27 A. cilicica cilicica 431 Tomb U-j 582 Agatharchides 162 A. cilicica isaurica 431 Cemetery U 79 agathic acid 453 A. nordmanniana 431 Abyssinia 46 Agathis 453, 464 abietane 445, 454 acacia 91, 148, 305, 335–6, 335, 344, 367, 487, Agricultural Museum, Dokki (Cairo) 558, 559, abietic acid 445, 450, 453 489 564, 632, 634, 666 abrasive 329, 356 Acacia 335, 476–7, 488, 491, 586 agriculture 228, 247, 341, 344, 391, 505, Abrak 148 A. albida 335, 477 506, 510, 515, 517, 521, 526, 528, 569, Abri-Delgo Reach 323 A. arabica 477 583, 584, 609, 615, 616, 617, 628, 637, absorption spectrophotometry 500 A. arabica var. adansoniana 477 647, 656 Abu (Elephantine) 323 A. farnesiana 477 agrimi 327 Abu Aggag formation 54, 55 A. nilotica 279, 335, 354, 367, 477, 488 A Group 323 Abu Ghalib 541 A. nilotica leiocarpa 477 Ahmose (Amarna oªcial) 115 Abu Gurob 410 A. -
The Ancient Egyptian Books of the Earth Wilbour Studies in Egypt and Ancient Western Asia
THE ANCIEN THE Collections of scenes and texts designated variously as the “Book of the Earth,” “Creation of the Solar Disc,” and “Book of Aker” were inscribed on the walls of royal sarcophagus chambers throughout Egypt’s Ramesside period (Dynasties 19–20). This material illustrated discrete episodes from the The Ancient Egyptian nocturnal voyage of the sun god, which functioned as a model for the resurrection of the deceased T king. These earliest “Books of the Earth” employed mostly ad hoc arrangements of scenes, united E by shared elements of iconography, an overarching, bipartite symmetry of composition, and their GYP Books of the Earth frequent pairing with representations of the double sky overhead. From the Twenty-First Dynasty and later, selections of programmatic tableaux were adapted for use in private mortuary contexts, T I often in conjunction with innovative or previously unattested annotations. The present study collects A and analyzes all currently known Book of the Earth material, including discussions of iconography, BOOKSN OF by Joshua Aaron Roberson grammar, orthography, and architectural setting. Joshua Aaron Roberson is an Assistant Professor in the Department of History, Camden County College. Blackwood, NJ. He has worked as an epigrapher and sigillographer with the University of Pennsylvania expeditions to Saqqara and Abydos and as a sigillographer for the French-Egyptian expedition to the Opet temple at Karnak. He earned his PhD in Egyptology from the University of Pennsylvania. T HE HE EA R T H Joshua Aaron Aaron Joshua Wilbour Studies R o berson Brown University Wilbour Studies in Egypt and Ancient Western Asia, 1 Department of Egyptology and Ancient Western Asian Studies LOCKWOOD PRESS www.lockwoodpress.com LOCKWOOD PRESS Wilbour_cover_template.indd 1 1/27/12 10:24 AM The Ancient Egyptian Books of the Earth Wilbour Studies in Egypt and Ancient Western Asia Series Editors James P. -
On the Modeling of Air Flow in the Tombs of the Valley of Kings
cs: O ani pe ch n e A c M c Khalil, Fluid Mech Open Acc 2017, 4:3 d e i s u s l F Fluid Mechanics: Open Access DOI: 10.4172/2476-2296.1000166 ISSN: 2476-2296 Research Article Open Access On the Modeling of Air Flow in the Tombs of the Valley of Kings Essam E Khalil1,2* 1Chairman Arab HVAC Code Committee ASHRAE Director-At-Large, USA, Convenor ISO TC205 WG2, Co-Convenor ISO TC163 WG4, Deputy Director (International) AIAA, USA 2DIC, Professor of Mechanical Engineering, Cairo University, Cairo, Egypt Abstract The tombs of the kings in Valley of the Kings, Luxor, are considered to be one of the tourism industry’s bases in Egypt due to their uniqueness all over the world. Hence, they should be preserved from the different factors that might cause harm for their wall paintings. One of these factors is the excessive relative humidity as it increases the bacteria and fungus activity inside the tomb in addition to its effect on the mechanical and physical properties of materials. This chapter describes the Research work to design ventilation systems to some of these important tombs. The chapter aims to investigate, design, and implement controlled climate to the tombs of the valley of kings with complete monitoring of air properties, temperature, relative humidity and carbon oxides and air quality parameters mechanical distributions inside selected tombs of the valley of the kings that are open for visitors. A complete climate control and monitoring of air will be effected with the aid of a mechanical ventilation system extracting air at designated locations in the wooden raised floor of the tombs. -
Heteromultimeric Potassium Channels Formed by Members of the Kv2 Subfamily
The Journal of Neuroscience, December 1, 1998, 18(23):9585–9593 Heteromultimeric Potassium Channels Formed by Members of the Kv2 Subfamily Judith T. Blaine and Angeles B. Ribera Department of Physiology and Biophysics, University of Colorado Health Sciences Center, Denver, Colorado 80262 Four a-subunits are thought to coassemble and form a voltage- timers, we developed a dominant-negative mutant Kv2.2 sub- a dependent potassium (Kv ) channel. Kv -subunits belong to unit to act as a molecular poison of Kv2 subunit-containing one of four major subfamilies (Kv1, Kv2, Kv3, Kv4). Within a channels. The dominant-negative Kv2.2 suppresses formation subfamily up to eight different genetic isotypes exist (e.g., of functional channels when it is coexpressed in oocytes with Kv1.1, Kv1.2). Different isotypes within the Kv1 or Kv3 subfamily either wild-type Kv2.2 or Kv2.1 subunits. These results indicate coassemble. It is not known, however, whether the only two that Kv2.1 and Kv2.2 subunits are capable of heteromultimer- members of the vertebrate Kv2 subfamily identified thus far, ization. Thus, in native cells either Kv2.1 and Kv2.2 subunits are Kv2.1 and Kv2.2, heteromultimerize. This might account for the targeted at an early stage to different biosynthetic compart- lack of detection of heteromultimeric Kv2 channels in situ de- ments or heteromultimerization otherwise is inhibited. spite the coexpression of Kv2.1 and Kv2.2 mRNAs within the Key words: potassium channels; heteromultimers; coassem- same cell. To probe whether Kv2 isotypes can form heteromul- bly; Kv2; dominant-negative mutant; Xenopus Voltage-dependent potassium (Kv ) channels play many crucial units do not colocalize, although in certain neurons their encoded roles in neuronal function. -
Depictions of the Sky–Goddess Nut in Three Royal Tombs of the New Kingdom and Her Relation to the Milky Way*
COSMIC SPACE AND ARCHETYPAL TIME: DEPICTIONS OF THE SKY–GODDESS NUT IN THREE ROYAL TOMBS OF THE NEW KINGDOM AND HER RELATION TO THE MILKY WAY* Amanda–Alice Maravelia ABSTRACT Nut was considered the sky–goddess par excellence in ancient Egypt. Unlike other Mediterranean civilizations the sky was conceived as a female divinity by the Nile dwel- lers. She was thought of as an amniotic cocoon inside which the pharaohs would regain life and immortality in the hereafter. Hence, being perceived as a Universal Mother ar- chetype, she would bestow rebirth in the afterlife to the dead kings, who were conside- red her children. In her virtual identification with the sarcophagus, Nut was considered a nursery and nurse, and was depicted inside sarcophagi and anthropoid coffins alike, stretching her body protectively over the corpse of the deceased. Joining with her would mean return to the womb of eternal space–time continuum and passing victoriously through immortality. The mural and ceiling paintings of the sky–goddess Nut in some royal tombs of the NK in the Valley of the Kings are renowned for their chromatic forms (mainly blue for the sky, golden yellow for the stars and red for the solar disc), as well as for their religiously æsthetic conceptions (cosmogony, theogony and their artistic li- turgical expression). In this paper we discuss the relation between cosmic space and ar- chetypal time, as those were viewed under the prism of ancient Egyptian mythological perceptions and were represented by the former depictions of Nut. The tombs of Phara- ohs Ramesses IV, Ramesses VI and Ramesses IX are examined. -
Risk Assessment of Flash Floods in the Valley of the Kings, Egypt
京都大学防災研究所年報 第 60 号 B 平成 29 年 DPRI Annuals, No. 60 B, 2017 Risk Assessment of Flash Floods in the Valley of the Kings, Egypt Yusuke OGISO(1), Tetsuya SUMI, Sameh KANTOUSH, Mohammed SABER and Mohammed ABDEL-FATTAH(1) (1) Graduate School of Engineering, Kyoto University Synopsis Flash floods unavoidably affect various archaeological sites in Egypt, through increased frequency and severity of extreme events. The Valley of the Kings (KV) is a UNESCO World Heritage site with more than thirty opened tombs. Recently, most of these tombs have been damaged and inundated after 1994 flood. Therefore, KV mitigation strategy has been proposed and implemented with low protection wall surrounding tombs. The present study focuses on the evaluation and risk assessment of the current mitigation measures especially under extreme flood events. Two dimensional hydrodynamic model combined with rainfall runoff modeling by using TELEMAC-2D to simulate the present situation without protection wall and determine the risk of 1994 flood. The results revealed that the current mitigation measures are not efficient. Based on the simulation scenarios, risk of flash floods is assessed, and the more efficient mitigation measurements are proposed. Keywords: Flash floods, The Valley of the Kings, TELEMAC-2D, Mitigation measures 1. Introduction Recently, most of these tombs have been damaged and inundated after 1994 flood. In response to this Egypt is one of arid and semiarid Arabian flood event, the American Research Center in Egypt countries that faces flash floods in the coastal and (ARCE) hired an interdisciplinary team of Nile wadi systems. Wadi is a dry riverbed that can consultants to prepare a flood-protection plan. -
ABSTRACT Carl Nicholas Reeves STUDIES in the ARCHAEOLOGY
ABSTRACT Carl Nicholas Reeves STUDIES IN THE ARCHAEOLOGY OF THE VALLEY OF THE KINGS, with particular reference to tomb robbery and the caching of the royal mummies This study considers the physical evidence for tomb robbery on the Theban west bank, and its resultant effects, during the New Kingdom and Third Intermediate Period. Each tomb and deposit known from the Valley of the Kings is examined in detail, with the aims of establishing the archaeological context of each find and, wherever possible, isolating and comparing the evidence for post-interment activity. The archaeological and documentary evidence pertaining to the royal caches from Deir el-Bahri, the tomb of Amenophis II and elsewhere is drawn together, and from an analysis of this material it is possible to suggest the routes by which the mummies arrived at their final destinations. Large-scale tomb robbery is shown to have been a relatively uncommon phenomenon, confined to periods of political and economic instability. The caching of the royal mummies may be seen as a direct consequence of the tomb robberies of the late New Kingdom and the subsequent abandonment of the necropolis by Ramesses XI. Associated with the evacuation of the Valley tombs may be discerned an official dismantling of the burials and a re-absorption into the economy of the precious commodities there interred. STUDIES IN THE ARCHAEOLOGY OF THE VALLEY OF THE KINGS, with particular reference to tomb robbery and the caching of the royal mummies (Volumes I—II) Volume I: Text by Carl Nicholas Reeves Thesis submitted for the degree of Doctor of Philosophy School of Oriental Studies University of Durham 1984 The copyright of this thesis rests with the author.