Epigenetic Regulators of White Adipocyte Browning
Total Page:16
File Type:pdf, Size:1020Kb
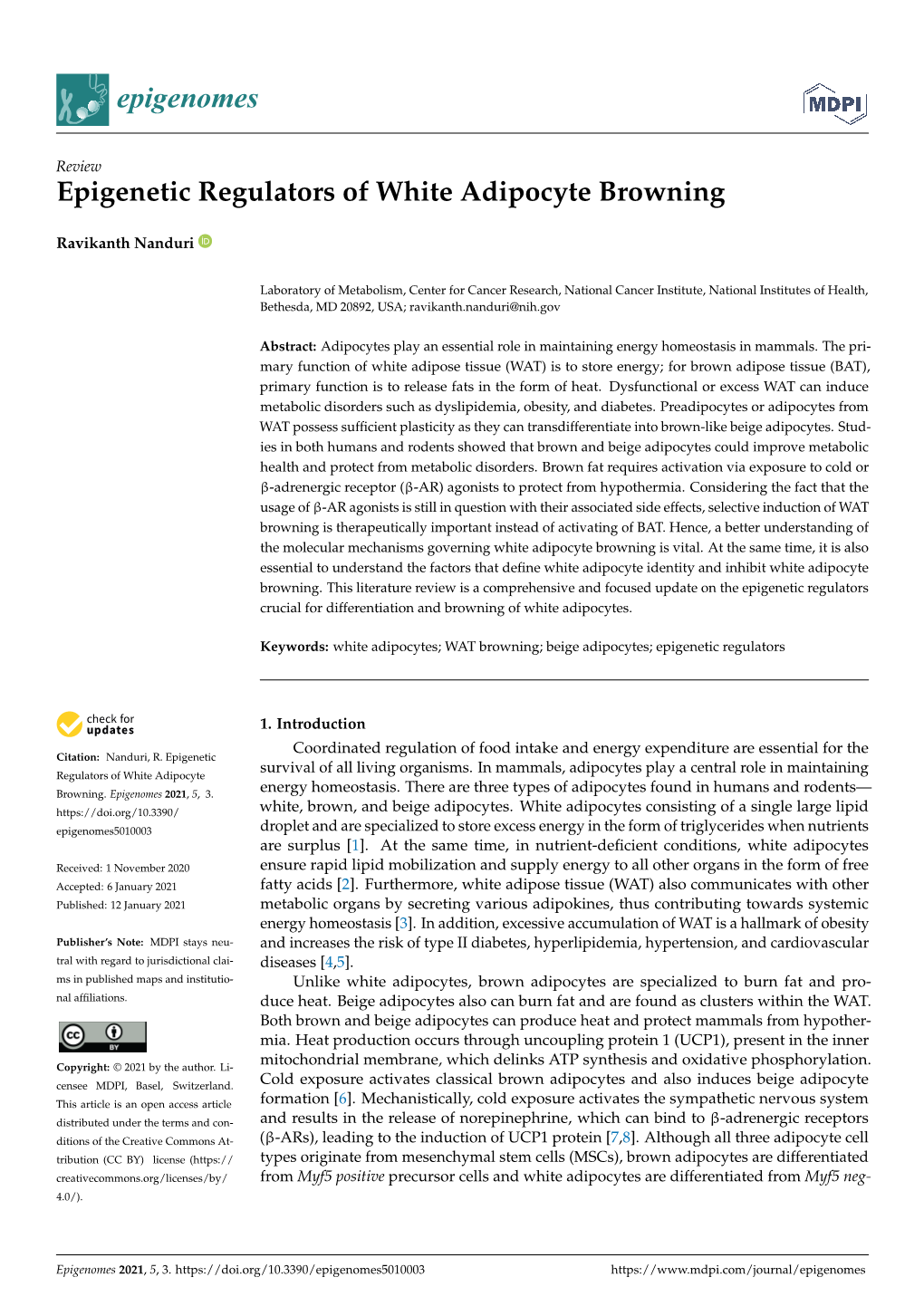
Load more
Recommended publications
-
Four Enzymes Cooperate to Displace Histone H1 During the First Minute of Hormonal Gene Activation
Downloaded from genesdev.cshlp.org on September 26, 2021 - Published by Cold Spring Harbor Laboratory Press Four enzymes cooperate to displace histone H1 during the first minute of hormonal gene activation Guillermo Pablo Vicent,1,2 A. Silvina Nacht,1 Jofre Font-Mateu, Giancarlo Castellano, Laura Gaveglia, Cecilia Ballare´, and Miguel Beato Centre de Regulacio´ Geno` mica (CRG), Universitat Pompeu Fabra (UPF), E-08003 Barcelona, Spain Gene regulation by external signals requires access of transcription factors to DNA sequences of target genes, which is limited by the compaction of DNA in chromatin. Although we have gained insight into how core histones and their modifications influence this process, the role of linker histones remains unclear. Here we show that, within the first minute of progesterone action, a complex cooperation between different enzymes acting on chromatin mediates histone H1 displacement as a requisite for gene induction and cell proliferation. First, activated progesterone receptor (PR) recruits the chromatin remodeling complexes NURF and ASCOM (ASC-2 [activating signal cointegrator-2] complex) to hormone target genes. The trimethylation of histone H3 at Lys 4 by the MLL2/MLL3 subunits of ASCOM, enhanced by the hormone-induced displacement of the H3K4 demethylase KDM5B, stabilizes NURF binding. NURF facilitates the PR-mediated recruitment of Cdk2/CyclinA, which is required for histone H1 displacement. Cooperation of ATP-dependent remodeling, histone methylation, and kinase activation, followed by H1 displacement, is a prerequisite for the subsequent displacement of histone H2A/H2B catalyzed by PCAF and BAF. Chromatin immunoprecipitation (ChIP) and sequencing (ChIP-seq) and expression arrays show that H1 displacement is required for hormone induction of most hormone target genes, some of which are involved in cell proliferation. -
Epigenetic Regulation of Muscle Stem Cell Expansion
Epigenetic regulation of muscle stem cell expansion INAUGURAL-DISSERTATION zur Erlangung des Doktorgrades der Naturwissenschaften - Doctor rerum naturalium - (Dr. rer. nat.) vorgelegt dem Fachbereich für Biologie und Chemie (FB 08) der Justus-Liebig-Universität Gießen eingereicht von Ting Zhang Gießen, 2015 Die vorliegende Arbeit wurde am Max-Planck-Institut für Herz- und Lungenforschung, W.G. Kerckhoff-Institut in Bad Nauheim angefertigt. Erstgutachter: Prof. Dr. Dr. Thomas Braun Abteilung Entwicklung und Umbau des Herzens Max-Planck-Institut für Herz- und Lungenforschung Ludwigstraße 43, 61231 Bad Nauheim Zweitgutachter: Prof. Dr. Lienhard Schmitz Biochemisches Institut Fachbereich Medizin Justus-Liebig-Universität Gießen Friedrichstrasse 24, 35392 Giessen Disputation am 10.Juni 2015 EIDESSTATTLICHE ERKLÄRUNG „Ich erkläre: Ich habe die vorgelegte Dissertation selbständig und ohne unerlaubte fremde Hilfe und nur mit den Hilfen angefertigt, die ich in der Dissertation angegeben habe. Alle Textstellen, die wörtlich oder sinngemäß aus veröffentlichten Schriften entnommen sind, und alle Angaben, die auf mündlichen Auskünften beruhen, sind als solche kenntlich gemacht. Bei den von mir durchgeführten und in der Dissertation erwähnten Untersuchungen habe ich die Grundsätze guter wissenschaftlicher Praxis, wie sie in der „Satzung der Justus-Liebig-Universität Gießen zur Sicherung guter wissenschaftlicher Praxis“ niedergelegt sind, eingehalten.“ Bad Nauheim, den ZUSAMMENFASSUNG ZUSAMMENFASSUNG Stammzellen der adulten Skelettmuskulatur, auch als -
Subcloning, Expression, and Enzymatic Study of PRMT5
Georgia State University ScholarWorks @ Georgia State University Biology Theses Department of Biology Summer 7-12-2010 Subcloning, Expression, and Enzymatic Study of PRMT5 Ran Guo Georgia State University Follow this and additional works at: https://scholarworks.gsu.edu/biology_theses Part of the Biology Commons Recommended Citation Guo, Ran, "Subcloning, Expression, and Enzymatic Study of PRMT5." Thesis, Georgia State University, 2010. https://scholarworks.gsu.edu/biology_theses/26 This Thesis is brought to you for free and open access by the Department of Biology at ScholarWorks @ Georgia State University. It has been accepted for inclusion in Biology Theses by an authorized administrator of ScholarWorks @ Georgia State University. For more information, please contact [email protected]. SUBCLONING, EXPRESSION, AND ENZYMATIC STUDY OF PRMT5 by RAN GUO Under the Direction of Yujun George Zheng ABSTRACT Protein arginine methyltransferases (PRMTs) mediate the transfer of methyl groups to arginine residues in histone and non-histone proteins. PRMT5 is an important member of PRMTs which symmetrically dimethylates arginine 8 in histone H3 (H3R8) and arginine 3 in histone H4 (H4R3). PRMT5 was reported to inhibit some tumor suppressors in leukemia and lymphoma cells and regulate p53 gene, through affecting the promoter of p53. Through methylation of H4R3, PRMT5 can recruit DNA-methyltransferase 3A (DNMT3A) which regulates gene transcription. All the above suggest that PRMT5 has an important function of suppressing cell apoptosis and is a potential anticancer target. Currently, the enzymatic activities of PRMT5 are not clearly understood. In our study, we improved the protein expression methodology and greatly enhanced the yield and quality of the recombinant PRMT5. -
Inferring Causal Relationships Among Different Histone Modifications and Gene Expression
Downloaded from genome.cshlp.org on September 28, 2021 - Published by Cold Spring Harbor Laboratory Press Inferring Causal Relationships among Different Histone Modifications and Gene Expression Hong Yu*, Shanshan Zhu*, Bing Zhou*, Huiling Xue and Jing-Dong J. Han Chinese Academy of Sciences Key Laboratory of Molecular Developmental Biology, Center for Molecular Systems Biology, Institute of Genetics and Developmental Biology, Chinese Academy of Sciences, Datun Road, Beijing, 100101, China * These authors contributed equally to this work. Correspondence should be addressed to J.-D.J.H ([email protected]). Running title: histone modification and gene expression network Keywords: histone modification, gene expression, Bayesian network, causal relationship, epigenetics 1 Downloaded from genome.cshlp.org on September 28, 2021 - Published by Cold Spring Harbor Laboratory Press Abstract Histone modifications are major epigenetic factors regulating gene expression. They play important roles in maintaining stem cell pluripotency and in cancer pathogenesis. Different modifications may combine to form complex ‘histone codes’. Recent high throughput technologies, such as ‘ChIP-chip’ and ‘ChIP-seq’, have generated high resolution maps for many histone modifications on the human genome. Here we use these maps to build a Bayesian network to infer causal and combinatorial relationships among histone modifications and gene expression. A pilot network derived by the same method among polycomb group (PcG) genes and H3K27 trimethylation is accurately supported by current literature. Our unbiased network model among histone modifications is also well supported by cross validation results. It not only confirmed already known relationships, such as those of H3K27me3 to gene silencing, H3K4me3 to gene activation, and the effect of bivalent modification of both H3K4me3 and H3K27me3, but also identified many other relationships that may predict new epigenetic interactions important in epigenetic gene regulation. -
Variable Clinical Expression in Patients with a Germline MEN1 Disease Gene Mutation: Clues to a Genotype–Phenotype Correlation
CLINICS 2012;67(S1):49-56 DOI:10.6061/clinics/2012(Sup01)10 REVIEW Variable clinical expression in patients with a germline MEN1 disease gene mutation: clues to a genotype–phenotype correlation Cornelis J. Lips,I Koen M. Dreijerink,I Jo W. Ho¨ ppenerII I University Medical Center Utrecht, Department of Internal Medicine & Endocrinology, Utrecht, The Netherlands. II University Medical Center Utrecht, Department of Metabolic & Endocrine Diseases, Utrecht, The Netherlands. Multiple endocrine neoplasia type 1 is an inherited endocrine tumor syndrome, predominantly characterized by tumors of the parathyroid glands, gastroenteropancreatic tumors, pituitary adenomas, adrenal adenomas, and neuroendocrine tumors of the thymus, lungs or stomach. Multiple endocrine neoplasia type 1 is caused by germline mutations of the multiple endocrine neoplasia type 1 tumor suppressor gene. The initial germline mutation, loss of the wild-type allele, and modifying genetic and possibly epigenetic and environmental events eventually result in multiple endocrine neoplasia type 1 tumors. Our understanding of the function of the multiple endocrine neoplasia type 1 gene product, menin, has increased significantly over the years. However, to date, no clear genotype– phenotype correlation has been established. In this review we discuss reports on exceptional clinical presentations of multiple endocrine neoplasia type 1, which may provide more insight into the pathogenesis of this disorder and offer clues for a possible genotype–phenotype correlation. KEYWORDS: Multiple Endocrine Neoplasia type 1; MEN1; Menin; Genotype–Phenotype Correlation; Clinical Expression. Lips CJ, Dreijerink KM, Ho¨ ppener JW. Variable clinical expression in patients with a germline MEN1 disease gene mutation: clues to a genotype– phenotype correlation. Clinics. 2012;67(S1):49-56. -
Anti-H3r2me2(Asym) Antibody
FOR RESEARCH USE ONLY! 01/20 Anti-H3R2me2(asym) Antibody CATALOG NO.: A2025-100 (100 µl) BACKGROUND DESCRIPTION: Histones are basic nuclear proteins that are responsible for the nucleosome structure of the chromosomal fiber in eukaryotes. Nucleosomes consist of approximately 146 bp of DNA wrapped around a histone octamer composed of pairs of each of the four core histones (H2A, H2B, H3, and H4). The chromatin fiber is further compacted through the interaction of a linker histone, H1, with the DNA between the nucleosomes to form higher order chromatin structures. This gene is intronless and encodes a replication-dependent histone that is a member of the histone H3 family. Transcripts from this gene lack poly A tails; instead, they contain a palindromic termination element. This gene is located separately from the other H3 genes that are in the histone gene cluster on chromosome 6p22-p21.3. H3.4; H3/g; H3FT; H3t; HIST3H3; Histone H3; HIST1H3A ALTERNATE NAMES: ANTIBODY TYPE: Polyclonal HOST/ISOTYPE: Rabbit / IgG IMMUNOGEN: A synthetic methylated peptide targeting residues around Arginine 2 of human Histone H3 MOLECULAR WEIGHT: 17 kDa PURIFICATION: Affinity purified FORM: Liquid FORMULATION: In PBS with 0.02% sodium azide, 50% glycerol, pH 7.3 SPECIES REACTIVITY: Human, Mouse, Rat STORAGE CONDITIONS: Store at -20ºC. Avoid freeze / thaw cycles APPLICATIONS AND USAGE: WB 1:500 - 1:2000, IHC 1:50 - 1:200, IF 1:50 - 1:200 Note: This information is only intended as a guide. The optimal dilutions must be determined by the user Western blot analysis of H3R2me2(asym) expression in Dot-blot analysis of methylation peptides using Anti- HeLa cells and H3 protein. -
Supplemental Materials ZNF281 Enhances Cardiac Reprogramming
Supplemental Materials ZNF281 enhances cardiac reprogramming by modulating cardiac and inflammatory gene expression Huanyu Zhou, Maria Gabriela Morales, Hisayuki Hashimoto, Matthew E. Dickson, Kunhua Song, Wenduo Ye, Min S. Kim, Hanspeter Niederstrasser, Zhaoning Wang, Beibei Chen, Bruce A. Posner, Rhonda Bassel-Duby and Eric N. Olson Supplemental Table 1; related to Figure 1. Supplemental Table 2; related to Figure 1. Supplemental Table 3; related to the “quantitative mRNA measurement” in Materials and Methods section. Supplemental Table 4; related to the “ChIP-seq, gene ontology and pathway analysis” and “RNA-seq” and gene ontology analysis” in Materials and Methods section. Supplemental Figure S1; related to Figure 1. Supplemental Figure S2; related to Figure 2. Supplemental Figure S3; related to Figure 3. Supplemental Figure S4; related to Figure 4. Supplemental Figure S5; related to Figure 6. Supplemental Table S1. Genes included in human retroviral ORF cDNA library. Gene Gene Gene Gene Gene Gene Gene Gene Symbol Symbol Symbol Symbol Symbol Symbol Symbol Symbol AATF BMP8A CEBPE CTNNB1 ESR2 GDF3 HOXA5 IL17D ADIPOQ BRPF1 CEBPG CUX1 ESRRA GDF6 HOXA6 IL17F ADNP BRPF3 CERS1 CX3CL1 ETS1 GIN1 HOXA7 IL18 AEBP1 BUD31 CERS2 CXCL10 ETS2 GLIS3 HOXB1 IL19 AFF4 C17ORF77 CERS4 CXCL11 ETV3 GMEB1 HOXB13 IL1A AHR C1QTNF4 CFL2 CXCL12 ETV7 GPBP1 HOXB5 IL1B AIMP1 C21ORF66 CHIA CXCL13 FAM3B GPER HOXB6 IL1F3 ALS2CR8 CBFA2T2 CIR1 CXCL14 FAM3D GPI HOXB7 IL1F5 ALX1 CBFA2T3 CITED1 CXCL16 FASLG GREM1 HOXB9 IL1F6 ARGFX CBFB CITED2 CXCL3 FBLN1 GREM2 HOXC4 IL1F7 -
UNIVERSITY of CALIFORNIA, IRVINE Combinatorial Regulation By
UNIVERSITY OF CALIFORNIA, IRVINE Combinatorial regulation by maternal transcription factors during activation of the endoderm gene regulatory network DISSERTATION submitted in partial satisfaction of the requirements for the degree of DOCTOR OF PHILOSOPHY in Biological Sciences by Kitt D. Paraiso Dissertation Committee: Professor Ken W.Y. Cho, Chair Associate Professor Olivier Cinquin Professor Thomas Schilling 2018 Chapter 4 © 2017 Elsevier Ltd. © 2018 Kitt D. Paraiso DEDICATION To the incredibly intelligent and talented people, who in one way or another, helped complete this thesis. ii TABLE OF CONTENTS Page LIST OF FIGURES vii LIST OF TABLES ix LIST OF ABBREVIATIONS X ACKNOWLEDGEMENTS xi CURRICULUM VITAE xii ABSTRACT OF THE DISSERTATION xiv CHAPTER 1: Maternal transcription factors during early endoderm formation in 1 Xenopus Transcription factors co-regulate in a cell type-specific manner 2 Otx1 is expressed in a variety of cell lineages 4 Maternal otx1 in the endodermal conteXt 5 Establishment of enhancers by maternal transcription factors 9 Uncovering the endodermal gene regulatory network 12 Zygotic genome activation and temporal control of gene eXpression 14 The role of maternal transcription factors in early development 18 References 19 CHAPTER 2: Assembly of maternal transcription factors initiates the emergence 26 of tissue-specific zygotic cis-regulatory regions Introduction 28 Identification of maternal vegetally-localized transcription factors 31 Vegt and OtX1 combinatorially regulate the endodermal 33 transcriptome iii -
An Evolutionary Conserved Region (ECR) in the Human Dopamine Receptor D4 Gene Supports Reporter Gene Expression in Primary Cultu
Paredes et al. BMC Neuroscience 2011, 12:46 http://www.biomedcentral.com/1471-2202/12/46 RESEARCHARTICLE Open Access An evolutionary conserved region (ECR) in the human dopamine receptor D4 gene supports reporter gene expression in primary cultures derived from the rat cortex Ursula M Paredes1,2, Vivien J Bubb1, Kate Haddley1, Gabriele A Macho1,3 and John P Quinn1* Abstract Background: Detecting functional variants contributing to diversity of behaviour is crucial for dissecting genetics of complex behaviours. At a molecular level, characterisation of variation in exons has been studied as they are easily identified in the current genome annotation although the functional consequences are less well understood; however, it has been difficult to prioritise regions of non-coding DNA in which genetic variation could also have significant functional consequences. Comparison of multiple vertebrate genomes has allowed the identification of non-coding evolutionary conserved regions (ECRs), in which the degree of conservation can be comparable with exonic regions suggesting functional significance. Results: We identified ECRs at the dopamine receptor D4 gene locus, an important gene for human behaviours. The most conserved non-coding ECR (D4ECR1) supported high reporter gene expression in primary cultures derived from neonate rat frontal cortex. Computer aided analysis of the sequence of the D4ECR1 indicated the potential transcription factors that could modulate its function. D4ECR1 contained multiple consensus sequences for binding the transcription factor Sp1, a factor previously implicated in DRD4 expression. Co-transfection experiments demonstrated that overexpression of Sp1 significantly decreased the activity of the D4ECR1 in vitro. Conclusion: Bioinformatic analysis complemented by functional analysis of the DRD4 gene locus has identified a) a strong enhancer that functions in neurons and b) a transcription factor that may modulate the function of that enhancer. -
The BTB-ZF Family of Transcription Factors: Key Regulators of Lineage
The BTB-ZF Family of Transcription Factors: Key Regulators of Lineage Commitment and Effector Function Development in the Immune System This information is current as of September 27, 2021. Aimee M. Beaulieu and Derek B. Sant'Angelo J Immunol 2011; 187:2841-2847; ; doi: 10.4049/jimmunol.1004006 http://www.jimmunol.org/content/187/6/2841 Downloaded from References This article cites 103 articles, 44 of which you can access for free at: http://www.jimmunol.org/content/187/6/2841.full#ref-list-1 http://www.jimmunol.org/ Why The JI? Submit online. • Rapid Reviews! 30 days* from submission to initial decision • No Triage! Every submission reviewed by practicing scientists • Fast Publication! 4 weeks from acceptance to publication by guest on September 27, 2021 *average Subscription Information about subscribing to The Journal of Immunology is online at: http://jimmunol.org/subscription Permissions Submit copyright permission requests at: http://www.aai.org/About/Publications/JI/copyright.html Email Alerts Receive free email-alerts when new articles cite this article. Sign up at: http://jimmunol.org/alerts The Journal of Immunology is published twice each month by The American Association of Immunologists, Inc., 1451 Rockville Pike, Suite 650, Rockville, MD 20852 Copyright © 2011 by The American Association of Immunologists, Inc. All rights reserved. Print ISSN: 0022-1767 Online ISSN: 1550-6606. The BTB-ZF Family of Transcription Factors: Key Regulators of Lineage Commitment and Effector Function Development in the Immune System Aimee M. Beaulieu* and Derek B. Sant’Angelo*,†,‡ Successful immunity depends upon the activity of mul- 1, -2, -4, -5, and -7 (1–11). -
Genome-Wide DNA Methylation Analysis of KRAS Mutant Cell Lines Ben Yi Tew1,5, Joel K
www.nature.com/scientificreports OPEN Genome-wide DNA methylation analysis of KRAS mutant cell lines Ben Yi Tew1,5, Joel K. Durand2,5, Kirsten L. Bryant2, Tikvah K. Hayes2, Sen Peng3, Nhan L. Tran4, Gerald C. Gooden1, David N. Buckley1, Channing J. Der2, Albert S. Baldwin2 ✉ & Bodour Salhia1 ✉ Oncogenic RAS mutations are associated with DNA methylation changes that alter gene expression to drive cancer. Recent studies suggest that DNA methylation changes may be stochastic in nature, while other groups propose distinct signaling pathways responsible for aberrant methylation. Better understanding of DNA methylation events associated with oncogenic KRAS expression could enhance therapeutic approaches. Here we analyzed the basal CpG methylation of 11 KRAS-mutant and dependent pancreatic cancer cell lines and observed strikingly similar methylation patterns. KRAS knockdown resulted in unique methylation changes with limited overlap between each cell line. In KRAS-mutant Pa16C pancreatic cancer cells, while KRAS knockdown resulted in over 8,000 diferentially methylated (DM) CpGs, treatment with the ERK1/2-selective inhibitor SCH772984 showed less than 40 DM CpGs, suggesting that ERK is not a broadly active driver of KRAS-associated DNA methylation. KRAS G12V overexpression in an isogenic lung model reveals >50,600 DM CpGs compared to non-transformed controls. In lung and pancreatic cells, gene ontology analyses of DM promoters show an enrichment for genes involved in diferentiation and development. Taken all together, KRAS-mediated DNA methylation are stochastic and independent of canonical downstream efector signaling. These epigenetically altered genes associated with KRAS expression could represent potential therapeutic targets in KRAS-driven cancer. Activating KRAS mutations can be found in nearly 25 percent of all cancers1. -
SUPPLEMENTARY MATERIAL Bone Morphogenetic Protein 4 Promotes
www.intjdevbiol.com doi: 10.1387/ijdb.160040mk SUPPLEMENTARY MATERIAL corresponding to: Bone morphogenetic protein 4 promotes craniofacial neural crest induction from human pluripotent stem cells SUMIYO MIMURA, MIKA SUGA, KAORI OKADA, MASAKI KINEHARA, HIROKI NIKAWA and MIHO K. FURUE* *Address correspondence to: Miho Kusuda Furue. Laboratory of Stem Cell Cultures, National Institutes of Biomedical Innovation, Health and Nutrition, 7-6-8, Saito-Asagi, Ibaraki, Osaka 567-0085, Japan. Tel: 81-72-641-9819. Fax: 81-72-641-9812. E-mail: [email protected] Full text for this paper is available at: http://dx.doi.org/10.1387/ijdb.160040mk TABLE S1 PRIMER LIST FOR QRT-PCR Gene forward reverse AP2α AATTTCTCAACCGACAACATT ATCTGTTTTGTAGCCAGGAGC CDX2 CTGGAGCTGGAGAAGGAGTTTC ATTTTAACCTGCCTCTCAGAGAGC DLX1 AGTTTGCAGTTGCAGGCTTT CCCTGCTTCATCAGCTTCTT FOXD3 CAGCGGTTCGGCGGGAGG TGAGTGAGAGGTTGTGGCGGATG GAPDH CAAAGTTGTCATGGATGACC CCATGGAGAAGGCTGGGG MSX1 GGATCAGACTTCGGAGAGTGAACT GCCTTCCCTTTAACCCTCACA NANOG TGAACCTCAGCTACAAACAG TGGTGGTAGGAAGAGTAAAG OCT4 GACAGGGGGAGGGGAGGAGCTAGG CTTCCCTCCAACCAGTTGCCCCAAA PAX3 TTGCAATGGCCTCTCAC AGGGGAGAGCGCGTAATC PAX6 GTCCATCTTTGCTTGGGAAA TAGCCAGGTTGCGAAGAACT p75 TCATCCCTGTCTATTGCTCCA TGTTCTGCTTGCAGCTGTTC SOX9 AATGGAGCAGCGAAATCAAC CAGAGAGATTTAGCACACTGATC SOX10 GACCAGTACCCGCACCTG CGCTTGTCACTTTCGTTCAG Suppl. Fig. S1. Comparison of the gene expression profiles of the ES cells and the cells induced by NC and NC-B condition. Scatter plots compares the normalized expression of every gene on the array (refer to Table S3). The central line